
Mechanical Properties of Ceramics and Composites
.pdf610 |
|
|
|
Chapter 10 |
TABLE 10.2 |
Representative |
|
|
|
Performance of Commercial Ceramics for |
||||
30 Caliber Armor Piercing Bullets |
|
|
|
|
|
|
|
|
|
Ceramic |
Density (gm/cc) |
V |
a |
|
|
|
|
50 |
|
B4C |
2.50 |
2090 |
|
|
SiC |
3.21 |
2000 |
|
|
Al2O3 |
3.96 |
1600 |
|
|
TiB2 |
4.50 |
1280 |
|
|
|
|
|
|
|
aRelative velocities at which half of the 30 caliber armor piercing (AP) projectiles penetrated through the test ceramic, i.e. higher values are better.
notch beam specimens was higher for 10 and 20 v/o whisker composites, crack extension after 500,000 cycles was respectively 2.2 and 2.4 times that of the matrix alone. On the other hand, the threshold stress range for fatigue crack initiation was 20% lower than for the matrix alone, and crack growth rates were smaller.
Limited ballistic testing of ceramic composites has been conducted, much of which is probably in classified literature. However, preliminary testing of reaction hot pressed composites during exploratory development [18,36] showed intermediate levels of performance (compare Tables 10.1 and 10.2). Also note that comparative testing of a commercial ZTA ( 30% ZrO2) gave lower 30 caliber AP V50 values of 1375. Further, DU (depleted uranium) penetrator tests of the reaction hot pressed AlN-SiC of Table 1 showed residual penetration the same as for commercial AlN, which approaches, but is poorer than, the performance of other commercial ballistic ceramics.
C.Erosion and Wear
Breder et al. [37] showed that erosion of Al2O3-ZrO2 by sharp airborne abrasive particles followed equations similar to Eq. (5.1) but with greater dependence on toughness. Residual strengths after erosion were an inverse function of particle kinetic energy.
Wada et al. [38,39] reported that HV (500 gm) of Al2O3 showed no increase from 19 GPa at 0 v/o SiC to 10 v/o α-SiC particles ( 1 m) or whiskers and then increased respectively to 25 and 28 GPa at 30 v/o, while
(I) toughness increased from 4.8 to 5.4 MPa·m1/2 over the range 0–30 v/o SiC. Erosion rates for normal impacts of Al2O3 and SiC particles ( 500 m) entrained in an air stream giving particle velocities of 250–300 m/sec were nearly twice as high with SiC versus Al2O3 particles and decreased with in-
Composite Particle and Grain Effects |
611 |
creases in both target hardness and toughness, though the correlation with the latter was more scattered. Similarly Wada and Watanabe [40] showed that such particle erosion resistance of Al2O3-TiC was higher than that of Al2O3, Al2O3- SiC, or Si3N4.
Yamada et al. [41] showed similar increases in HV (4.9 N) and somewhat lower increases in (I) toughness on addition of up to 40 v/o SiC particles ( 0.7m) and improved resistance to abrasion with Al2O3 or SiC particles, with the resistance of the Al2O3-SiC exceeding that of either Al2O3 or SiC by themselves when the SiC content was > 20 v/o. The abrasion rate was inversely dependent on H but was independent of toughness.
He et al. [42] reported beneficial effects of Al2O3-ZrO2 composites on the transition from a mainly plastic deformation to a mainly fracture dominated mechanism of sliding wear using a test with a Si3N4 ball on three flat composite specimens. The load for this sliding wear transition increased from 170 to 350 N as the composition changed from 5 to 20 v/o unstabilized ZrO2, with much of the effect attributed to reduction of the grain size of the Al2O3, especially of the largest grains. However, the lowest wear rate was found with 15 v/o ZrO2, showing additional benefits of the ZrO2, which was attributed to reduction of hardness and compressive surface stresses.
The sliding wear behavior of both a 5 a/o Y-TZP + 28 v/o Al2O3 and a ZTA composite (Al2O3+ 15 w/o, 10 v/o, ZrO2) were studied by He et al. [43]. They showed that increasing grain size in the former composite resulted in increasing wear due to increasing grain pullout attributed to increased ZrO2 transformation and resultant grain boundary weakening. At finer grain size where no transformation occurred, the former composite gave a fourfold lower wear rate compared to pure Y-TZP of the same grain size, which was attributed to the higher hardness from the Al2O3 addition. Little grain pullout and no microcracking was observed in the finer grain composites, whose wear characteristics were not impacted by sinter-forging of the bodies. The ZTA composite showed opposite dependence on G, i.e. increased wear resistance as G increased, which was attributed to increased compressive surface stresses from transformation of ZrO2, and had wear rates reduced twoor threefold by sinter forging with the same resultant grain size.
Wu et al. [44] measured diamond pin on disk (POD), i.e. scratch hardnesses of several ceramic composites versus several monolithic hard ceramics, e.g. constituents of the composites, using a 90° conical diamond pin (0.08 m radius) with loads of 0.5, 1, or 1.5 kg with the disk specimens rotating so the pin scribed a circle 1 cm radius at a speed of 1 cm/sec. Results showed poorer performance for Al2O3 with 30% ZrO2 or 15 w/o SiC particles, but superior performance for Al2O3 with 10% ZrO2 or 30% SiC whiskers (Fig. 10.3). The poor performance of the Al2O3 with 15% SiC was attributed to the large SiC particle size (> 40 m), while the good performance for the commercial
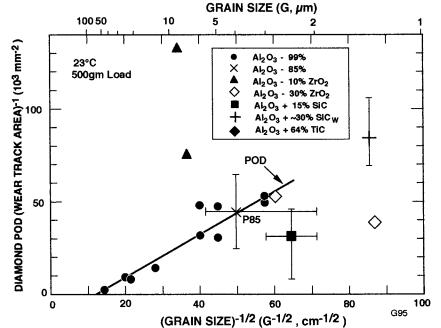
612 |
Chapter 10 |
FIGURE 10.3 Wear resistance from diamond pin on disk wear test of some ceramic composites versus the inverse square root of their matrix grain size compared with data for alumina bodies. Note (1) compositions in v/o, (2) composites can have similar, or better, wear resistance than comparable grain size alumina bodies, and (3) poorer performance was associated with bodies having large particles of SiC or microcracking (Al2O3-30% ZrO2). (From Ref. 44. Published with permission of Ceramic Engineering and Science Proceedings.)
Al2O3+ 30% SiC whiskers was also approximately consistent with expectations for Al2O3 of the same G as in the composite matrix (see Fig. 8.14 for a fracture photo of this material). Reaction hot pressed composites of Al2O3 with 34 or 47% SiC, 47 or 64 v/o TiC, or 71.4 v/o TiB2 all with G and D a few microns, all gave low POD wear rates, e.g. values of 20–90 on the wear scale of Figure 10.3, indicating superior performance relative to pure Al2O3 of the same G. Fig. 4 shows that these dense fine-grain composites showed very similar appearances of surface plastic deformation in the wear tracks as dense fine-grain monolithic bodies, e.g. of alumina.
Krell and Klaffke [14] reported that their fine G ( 1.5 m) Al2O3-35 v/o TiC had a friction coefficient of 0.3–0.5 that was higher, e.g. by 20–30%, and had more dependence on the H2O level in the tests than for similar G Al2O3. The

Composite Particle and Grain Effects |
613 |
A
100 M
B
20 M
FIGURE 10.4 SEM photos of diamond pin on disk wear tracks on an Al2O3-64 v/o TiC reaction processed composite. (A) lower magnification and (B) higher magnification. Note parallel striations indicative of plastic flow and irregular transverse markings indicative of interruptions of this flow (compare to Figs. 5.13, 5.14). (From Ref. 44. Published with permission of Ceramic Engineering and Science Proceedings.)
614 |
|
|
|
|
Chapter 10 |
TABLE 10.3 Relative Abrasive Wear Performance of Various Commercial Ceramics |
|||||
Versus Commercial 85% Alumina |
|
|
|
||
|
|
|
|
|
|
Materiala (H, GPa) |
Grit blastb |
Slurryc |
Taberd |
Millinge |
|
|
|
|
|
|
|
99% Al2O3 |
(15.2) |
1.10 |
1.36 |
1.89 |
10f |
96% Al2O3 |
(12.6) |
1.06 |
— |
1.57 |
5.6 |
85% Al2O3 |
(10) |
1.0 |
1.0 |
1.0 |
1.0 |
ZTA (15.7) |
0.51 |
0.37 |
0.37 |
2.4 |
|
TZP (11.5) |
0.38 |
0.22 |
0.22 |
— |
|
Si3N4 (16.5) |
0.27 |
— |
0.39 |
— |
|
|
|
|
|
|
|
aCommercial materials and data from Diamonite Products. All values normalized to 85%
Al2O3 = 1.
bMeasured as the weight loss of plate specimens after a fixed exposure to a continuous grit blasting normal to the surface with 36 grit SiC abrasive.
cMeasured as the weight loss of plate specimens after a fixed exposure to continuous rotation in a fixed sand slurry composition.
dMeasured as the weight loss after a fixed number of rotations of plate specimens under two diamond abrasive wheels 1/2 inch wide.
eMeasured as the weight loss after a fixed exposure of rod specimens to each other with a specific charge of water and rods in a mill (whose size is scaled with rod size). For the tests here 1/4 inch dia. rods were used in a 5 liter mill.
fApproximate value, since a somewhat different firing schedule with resultant increased G was used for this test.
Source: Courtesy of J. Chakraverty of Diamonite Products.
oscillating wear rate of the composite was also close to but somewhat above that of the comparable alumina.
Tests of commercial ceramics using various industrial screening tests for different wear applications generally show ZTA (Al2O3+ 30 v/o ZrO2) to be better than commercial aluminas but not quite as good as TZP or Si3N4 (Table 10.3). However, there are some exceptions to this, e.g. ZTA was not superior to 85% alumina in ball milling tests.
IV. DISCUSSION AND SUMMARY
There is more data for hardness than for other properties of this chapter. This data shows that a linear dependence of hardness (H) per Eq. (10.1) is frequently suitable for composites, but that effects of the dispersed phase reducing the matrix grain size [13,17,19] need to be accounted for, and more complex behavior can occur, e.g. Fig. 1, due to reactions, heterogeneities, changes in orientation, etc. Some effect of second phase dimensions is indicated by lower hardness with larger SiC platelets in Si3N4 [20]. Definitive effects of interlaminar or rod spacings (λ) in directionally solidified eutectics was shown, i.e. H increased as λ-1/2
Composite Particle and Grain Effects |
615 |
increased until the eutectic structure started to break down. This λ-1/2 dependence of H is analogous to a G -1/2 dependence in monolithic ceramics, which is logical since λ simply measures the dimension of the matrix separating the aligned lamelli or rods of the eutectic just as G measures not only the grain dimension but also the separation between next nearest grains. Cracking in eutectics can also occur and reduce H [29] and is probably a factor in the H decreasing as the eutectic structure starts to break down.
Very little data on compressive strength and ballistic performance of composites exists; thus we miss a possible opportunity of using compressive testing as another probe to understand effects of microstructural stresses and microcracking. However, one set of considerable compressive testing [31] clearly shows the diversity and complexity of behavior that can occur in one common crystallized glass system (Fig. 10.2). Also, Simpson’s testing of his ceramic–metal composites made of particles of alternating laminated layers of metal and ceramic showing tensile and especially compressive strength increasing as the laminate particle size decreases [32] demonstrates another scale dimension that can impact strengths. Though no detailed studies of particle size effects of composites on their ballistic performance are available (and no definitive information exists on the G dependence of ballistic performance of monolithic ceramics, Chap. 5, Sec. III), preliminary testing of reaction processed ceramic composites showed potential for competitive behavior despite having almost no development (Tables 10.1, 10.2).
Though it is limited, there is more wear data for ceramic composites, which also shows potential for their use. Thus there is similar or greater wear resistance of Al2O3-based composites with similar fine matrix grain sizes to those of monolithic alumina bodies (Fig. 10.3), but again there is evidence that even limited microcracking from larger particles (SiC in Al2O3, Fig. 10.3) or compositional effect (Al2O3-30 v/o ZrO2) seriously limits wear resistance. Plastic deformation of composites with fine microstructures (Fig. 10.4) is indicated as for fine-grain-size monolithic ceramics. However, data is also needed on friction, which can be higher in some composites [14].
More limited data for abrasion and erosion of ceramic composites indicates performance similar to that for Eq. (5.1) [37], and that some composites, e.g. Al2O3 with fine SiC particles [41], can have promising resistance to damage.
REFERENCES
1.H. C. Lee and J. Gurland. Hardness and Deformation of Cemented Tungsten Carbide. Mat. Sci. Eng. 33:125–133, 1978.
2.N. Miyata and H. Jinno. Micromechanics Approach to the Indentation Hardness of Glass Matrix Particulate Composites. J. Mat. Sci. 17:547–557, 1982.
616 |
Chapter 10 |
3.J. D. French, H. M. Chan, M. P. Harmer, and G. A. Miller. Mechanical Properties
of Interpenetrating Microstructures: The Al2O3/c-ZrO2 System. J. Am. Cer. Soc. 75(2):418–423, 1992.
4.R. Roesky and J. R. Varner. Influence of Thermal History on the Crystallization Behavior and Hardness of a Glass-Ceramic. J. Am. Cer. Soc. 74(5):1129–1130, 1991.
5.A. J. Stryjak and P. W. McMillan. Microstructure and Properties of Transparent Glass-Ceramics. J. Mat. Sci. 13:1794–1804, 1978.
6.M. Tashiro and S. Sakka. Studies on the Mechanical Strength of the Photosensitive Opal Glass. J. Cer. Asn. Jpn. 68(6):72–77, 1960.
7.R. F. Cook, S. W. Freiman, and T. L. Baker. Effect of Microstructure on Reliability Predictions for Glass Ceramics. Mat. Sci. Eng. 77:199–212, 1986.
8.I. W. Donald and R. A. McCurrie. Microstructure and Indentation Hardness of an
MgO-LiO2-Al2O3-SiO2-TiO2 Glass-Ceramic. J. Am. Cer. Soc. 55(6):289–291, 1972.
9.W. D. Wolf, K. J. Vaidya, and L. F. Francis. Mechanical Properties and Failure Analysis of Alumina-Glass Dental Composites. J. Am. Cer. Soc. 79(7):1769–1776, 1996.
10.H. Ruf and A. G. Evans. Toughening by Monoclinic Zirconia. J. Am. Cer. Soc. 66(5):328–332, 1983.
11.M. Hirano and H. Inada. Fracture Toughness, Strength and Vickers Hardness of Yttria-Ceria-Doped Tetragonal Zirconia/Alumina Composites Fabricated by Hot Isostatic Pressing. J. Mat. Sci. 27:3511–3518, 1992.
12.H. J. Hwang and K. Niihara. Perovskite-Type BaTiO3 Ceramics Containing Particulate SiC. J. Mat. Sci. 33:549–558, 1998.
13.A. Nakahira, K. Niihara, and T. Hirai. Microstructural Properties of Al2O3-SiC Composites. Yogyo-Kyokai-Shi 94(8):767–772, 1986.
14.A. Krell and D. Klaffke. Effects of Grain Size and Humidity on Fretting Wear in
Fine-Grained Alumina, Al2O3/TiC, and Zirconia. J. Am. Cer. Soc. 79(5):1139–1146, 1996.
15.H. Endo, M. Ueki, and H. Kubo. Microstructure and Mechanical Properties of Hot Pressed SiC-TiC Composites. J. Mat. Sci. 26:3769–3774, 1991.
16.G. Sasaki, H. Nakase, K. Suganuma, T. Fujita, and K. Niihara. Mechanical Proper-
ties and Microstructure of Si3N4 Matrix Composite with Nano-Meter Scale SiC Particles. J. Cer. Soc. Jpn., Intl. Ed. 100:536–540, 1992.
17.A. K. Bhattacharya and J. J. Petrovic. Hardness and Fracture Toughness of SiC-
Particle-Reinforced MoSi2 Composites. J. Am. Cer. Soc. 74(10):2700–2703, 1991.
18.C. P. Cameron, J. H. Enloe, L. E. Dolhert, and R. W. Rice. A Comparison of Reaction vs. Conventionally Hot-Pressed Ceramic Composites. Cer. Eng. Sci. Proc. 11(9–10):1190–1202, 1990.
19.M. Landon and F. Thevenot. The SiC-AlN System: Influence of Elaboration Routes on the Solid Solution Formation and Its Mechanical Properties. Cer. Intl. 17:97–110, 1991.
Composite Particle and Grain Effects |
617 |
20.D. Baril, S. P. Tremblay, and M. Fiset. Silicon Carbide Platelet Reinforced Silicon Nitride Composites. J. Mat. Sci. 28:5486–5494, 1993.
21.M. Ashizuka, Y. Aimoto, and M. Watanabe. Mechanical Properties of SiC Whisker Reinforced Glass Ceramic Composites. J. Cer. Soc. Jpn., Intl. Ed. 97:783–789, 1989.
22.N. Tamari, H. Kobayashi, T. Tanaka, I. Kondoh, and S. Kose. Mechanical Proper-
ties of B4C-SiC Whisker Composite Ceramics. J. Jpn Cer. Soc., Intl. Ed. 98:1169–1173, 1990.
23.J. Dusza, D. Sajgalik, and M. Reece. Analysis of Si3N4+ -Si3N4 Whisker Ceramics. J. Mat. Sci. 26:6782–6788, 1991.
24.M. Nawa, T. Sekino, and K. Niihara. Fabrication and Mechanical Behavior of
Al2O3/Mo Nanocomposites Processing and Novel Interpenetrated Intragranular Microstructure. J. Mat. Sci. 29:3185–3192, 1994.
25.W. B. Chou, W. H. Tuan, and S. T. Chang. Preparation of NIAl Toughened Al2O3 by Vacuum Hot Pressing. Brit. Cer. Trans. 95(2):71–74, 1996.
26.F. L. Kennard, R. C. Bradt, and V. S. Stubican. Mechanical Properties of the Direc-
tionally Solidified MgO-MgAl2O4 Eutectic. J. Am. Cer. Soc. 59(3–4):160–163, 1976.
27.V. S. Stubican and R. C. Bradt. Eutectic Solidification in Ceramic Systems. Ann. Rev. Mat. Sci. 11:267–297, 1981.
28.V. S. Stubican, R. C. Bradt, F. L. Kennard, W. J. Minford, and C. C. Sorell. Ceramic Eutectic Composites. Tailoring Multiphase and Composite Ceramics, Materials Science Research 20 (R. E. Tressler, G. L. Messing, C. G. Pantano, and R. E. Newnham, eds.). Plenum Press, New York, 1986, pp. 103–113.
29.C. C. Sorell, V. S. Stubican, and R. C. Bradt. Mechanical Properties of ZrC-ZrB2 and ZrC-TiB2 Directionally Solidified Eutectics. J. Am. Cer. Soc. 69(4):317–321, 1986.
30.J. Echigoya, Y. Takabayashi, and H. Suto. Hardness and Fracture Toughness of Di-
rectionally Solidified Al2O3- ZrO2(Y2O3) Eutectics. J. Mat. Sci. Lett. 5:153–154, 1986.
31.C. A. May and A. K. U. Obi. Compressive Strength of Lithia-Silica Glass Ceramics. Mechanical Properties of Ceramics (2) (R. W. Davidge, ed.). Proc. Brit. Cer. Soc. 25:49–65, 5/1975.
32.A. R. B. Verma, V. S. R. Murthy, and G. S. Murty. Microstructure and Compressive Strength of SiC-Platelet-Reinforced Borosilicate Composites. J. Am. Cer. Soc. 78(10):2732–2736, 1995.
33.F. H. Simpson, Macrolaminate Particle Composite Material Development. Summary Report for Boeing Co. Work on US Navy Bureau of Weapons Contract No. 64-0194-f, 5/1965.
34.W. J. Knapp and F. R. Shanley. Process for Making Laminated Materials. US Patent 3,089,796, 5/1963.
35.S. Suresh, L. X. Han, and J. J. Petrovic. Fracture of Si3N4-SiC Whisker Composites Under Cyclic Loads. J. Am. Cer. Soc. 71(3):C–158–161, 1988.
36.R. W. Rice, C. P. Cameron, and P. L. Berneburg. Lower Cost Ceramic Armor Ma-
618 |
Chapter 10 |
terials. Proceedings Second Ballistics Symposium on Classified Topics, Johns Hopkins Applied Physics Lab. American Defense Preparedness Assn., 1992.
37.K. Breder, G. DePortu, J. E. Ritter, and D. D. Fabbriche. Erosion Damage and Strength Degradation of Zirconia-Toughened Alumina. J. Am. Cer. Soc. 71(9):770–775, 1988.
38.S. Wada, N. Watanabe, and T. Tani. Solid Particle Erosion of Brittle Materials (Part
6)—The Erosive Wear of Al2O3-SiC Composites. J. Jpn. Cer. Soc., Intl. Ed. 96:113–119, 1988.
39.S. Wada, N. Watanabe, and T. Tani. Solid Particle Erosion of Brittle Materials (Part
9)—The Erosive Wear of Al2O3-SiC Particle Composites. J. Jpn. Cer. Soc., Intl. Ed. 96:737–740, 1988.
40.S. Wada and N. Watanabe. Solid Particle Erosion of Brittle Materials (Part 7)— The Erosive Wear of Commercial Ceramic Tools. J. Jpn. Cer. Soc., Intl. Ed. 96:323–325, 1988.
41.K. Yamada, N. Kamiya, and S. Wada. Abrasive Wear of Al2O3-SiC Composites. J. Cer. Soc. Jpn., Intl. Ed. 99:797, 1991.
42.C. He, Y. S. Wang, J. S. Wallace, and S. M. Hsu. Effect of Microstructure on the Wear Transition of Zirconia-Toughened Alumina. Wear 162–164:314–321, 1993.
43.Y. J. He, A. J. A. Winnubst, A. J. Burggraaf, H. Verweij, P. G. T. Van der Varst, and
G. de With. Sliding Wear of ZrO2-Al2O3 Composite Ceramics. J. Eur. Cer. Soc. 17(11):1371–1380, 1997.
44.C. Cm. Wu, R. W. Rice, C. P. Cameron, L. E. Dolhert, J. H. Enloe, and J. Block.
Diamond Pin-on-Disk Wear of Al2O3 Matrix Composites and Nonoxides. Cer. Eng. Sci. Proc. 12(7–8):1485–1499, 1991.

11
Particle and Grain Effects on Mechanical Properties of Composites at Elevated Temperature
I.INTRODUCTION
This chapter addresses the effects of particle (and matrix grain) parameters on the mechanical properties of ceramic composites as a function of temperature, i.e. analogous to the treatment of grain effects on monolithic ceramics in Chapters 6 and 7. Again, the term particle is used in both a generic sense to include platelet, whisker, and fiber (e.g. in directionally solidified eutectics), and for actual particle composites. The primary particle parameters of interest besides composition are volume fraction (φ) and size as well as shape, and orientation. While the amount of desired information is often limited, some useful insight and guidance can be obtained. The focus is again more on changes in mechanical properties at more modest temperature where failure is primarily brittle fracture, in part to aid in understanding fracture at and near room temperature. Thus creep and related deformation are not a focus, but some results are noted, e.g. to emphasize the changes that such behavior often entails. However, thermal shock is addressed, and the limited but important data on thermal shock fatigue and the very limited data on related mechanical fatigue are addressed.
After a brief discussion of the limited theoretical background, the topics treated are elastic and related behavior, crack propagation and fracture energy or toughness, thermal shock and fatigue and related mechanical fatigue, tensile (flexure) strength, and hardness with compressive strength and related behavior.
619