
Yang Fluidization, Solids Handling, and Processing
.pdfHeat Transfer in Fluidized Beds 165
some correlation such as that of Yagi and Kunii (1957) be used to estimate the value kpa . In an alternate treatment, Botterill and Williams (1963) numerically solved the transient conduction problem for a single layer of closely packed spheres in a stagnant fluid at the heat transfer surface. They reported that for particles with diameters less than 200 μm, the solid temperature in the particles approached the temperature of the heat transfer surface in as little as 10 ms. This approach was later extended by Botterill et al. (1967) to multiple layers of particles close packed on the surface in a triangular lattice geometry. While such numerical analysis can represent the transient thermal process, it requires individual solutions for different particle material, particle size, and type of fluid. To alleviate this difficulty, a number of authors recognized that particles packed at a flat surface tend to have only point contacts so that an equivalent gas film separates the packed particles from the surface. Investigators who used this concept of a film resistance to modify the packet theory include Baskakov (1964), and Xavier and Davidson (1978). Gabor (1970) further extended this approach and modeled packets as alternating slabs of gas and solid. He reported that empirical agreement between model and experiment could be obtained by choosing a gas gap of 0.015 dp between particles and 0.0075 dp between the wall and the first particle layer. Kubie and Broughton (1975) suggested that this film resistance can be represented more realistically by Kunii and Smith’s relationship for effective thermal conductivity (1960) if the local void fraction is allowed to vary as a function of distance away from the heat transfer surface. For spherical particles, the local voidage varies with distance from the wall as a damped oscillation curve with minimum voidage at approximately one particle radius from the surface. This approach results in an effective thermal conductivity that varies with position, requiring a numerical solution of the transient conduction equation for packet heat transfer. Ozkaynak and Chen (1980) suggested a pragmatic simplification of Kubie and Broughton’s approach which removed the need for numerical computations. They suggested that the packet has an effective void fraction equivalent to that of a packed bed at a distance half of the thermal penetration depth from the wall. The penetration depth was defined as the distance where the packet attains 90% of the temperature difference between wall and bulk bed during its time of residence. For long contact times or small particles, where the penetration depth is more than two particle diameters, the average packet void fraction is taken to be that of a bulk packed bed. For large particles or

166 Fluidization, Solids Handling, and Processing
short residence times, where the penetration depth is less than two particle diameters, the effective void fraction is taken to be
|
|
æ |
y |
ö |
|
y |
|
|
∞ |
ç |
|
÷ |
|
|
|
Eq. (6) |
ε g, pa = ε g, pa - 3.04 log |
ç |
d p |
÷ |
for |
d p |
< 1 |
|
|
è |
ø |
|
|
where ε∞g,pa = void fraction of bulk packing. Using this effective void fraction, these authors were able to calculate packet conductivities using some model such as that of Kunii and Smith (1960) or Yagi and Kunii (1957), and to directly apply the packet model of Mickley and Fairbanks (Eq. 4). This modified packet theory was found to give good agreement with experimental measurements as shown in Fig. 7.
Figure 7. Comparison of data with packet theory, as modified by Ozkaynak and Chen (1980).
Heat Transfer in Fluidized Beds 167
The second approach assigns thermal resistance to a gaseous boundary layer at the heat transfer surface. The enhancement of heat transfer found in fluidized beds is then attributed to the scouring action of solid particles on the gas film, decreasing the “effective film thickness.” The early works of Leva et al. (1949), Dow and Jacob (1951), and Levenspiel and Walton (1954) utilized this approach. Models following this approach generally attempt to correlate a heat transfer Nusselt number in terms of the fluid Prandtl number and a modified Reynolds number with either the particle diameter or the tube diameter as the characteristic length scale. Examples are:
Leva correlation for vertical surfaces, for larger particles (1952),
Eq. (7) |
Nu = |
hc d p |
= 0.525 (Rep)0.75 |
||
|
k g |
||||
|
|
|
|
|
|
where |
Rep = |
|
d p |
ρg u |
|
|
μg |
||||
|
|
|
Vreedenberg correlation for horizontal tubes (1958),
|
|
hc D t |
|
|
|
|
æ |
|
ρ |
s |
ö0.3 |
æ |
μ g2 |
ö |
0.3 |
|
|
||||
Eq. (8) |
Nu = |
= 420 |
ç |
|
|
|
÷ |
ç |
|
|
|
÷ |
( |
Pr g |
)0.3 |
||||||
|
|
|
|
|
2 |
|
|
||||||||||||||
|
|
|
|
|
|
ç |
|
ρ g |
Ret ÷ |
ç |
|
3 ÷ |
|
|
|||||||
|
|
k g |
|
|
|
|
è |
|
ø è |
g ρ s |
d p ø |
|
|
|
|||||||
|
|
æ r |
|
|
|
ö |
|
|
|
|
|
|
|
|
|
|
|
||||
|
ç |
|
s |
|
|
|
÷ |
|
> 2250 |
|
|
|
|
|
|
|
|
||||
|
|
|
|
|
|
|
|
|
|
|
|
|
|
|
|||||||
|
|
for ç |
|
|
|
Ret ÷ |
|
|
|
|
|
|
|
|
|
||||||
|
|
è rg |
|
|
|
ø |
|
|
|
|
|
|
|
|
|
|
|
||||
where |
|
Ret |
= |
|
Dt |
|
ρ |
g |
|
u |
|
|
|
|
|
|
|
|
|||
|
|
|
|
|
|
|
|
|
|
|
|
|
|
|
|
|
|||||
|
|
|
|
μ g |
|
|
|
|
|
|
|
|
|
|
|
||||||
|
|
|
|
|
|
|
|
|
|
|
|
|
|
|
|
|
|

Heat Transfer in Fluidized Beds 169
Recently, Borodulya et al. (1989, 1991) described the gas film in terms of an effective conductivity divided by an effective conduction path length. Correlation of their model with experimental data gave the following equation,
|
|
|
0.1 |
æ |
ρ s |
ö014. |
æ Cs |
ö0.24 |
2/ 3 |
|
ε 2s/ 3 |
|
Eq. (9) |
Nu =0.74 |
Ar |
|
ç |
|
÷ |
ç |
|
÷ |
ε s |
+ 0.46RePr |
|
|
|
|
|
|||||||||
|
|
ç |
|
÷ |
ç |
|
÷ |
|
ε g |
|||
|
|
|
|
è |
ρ g ø |
èCg ø |
|
|
where the first term represents particle convection and the second term represents gas convection. Equation (9) is claimed to be good for the following ranges of parameters:
0.1≤ d p ≤ 4.0 mm
0.1≤ p ≤ 10.00 MPa
140 ≤ Ar ≤ 1.1×107
The correlation was tested against nine experimental sets of data with a mean-square error of 22%. Visser and Valk (1993) subsequently modified the particle convection term of the model of Borodulya et al. for low gas velocities. Their results indicated improved agreement for low velocity ranges.
In 1989 Molerus and Schweinzer (1989) presented an interesting variation of this approach. These investigators reasoned that the combined convective component of heat transfer is dominated by gas convection through the matrix of particles in the vicinity of the heat transfer surface, for beds of large particles and/or at increased system pressures. Noting that this same concept would hold for packed (fixed) beds, they then developed a nondimensional correlation which is valid for fixed beds as well as for fluidized beds of Geldart’s group D particles. The convective heat transfer Nusselt number was correlated to a nondimensional group which represents the pressure drop in the bed. In fixed bed regime, the Nusselt number varies as a function of Reynolds and Euler numbers. When the gas velocity exceeds that at minimum fluidization, the bed pressure drop and the convective Nusselt number becomes constant for a given type of particles, independent of gas velocity. In the fluidized regime, the correlation of
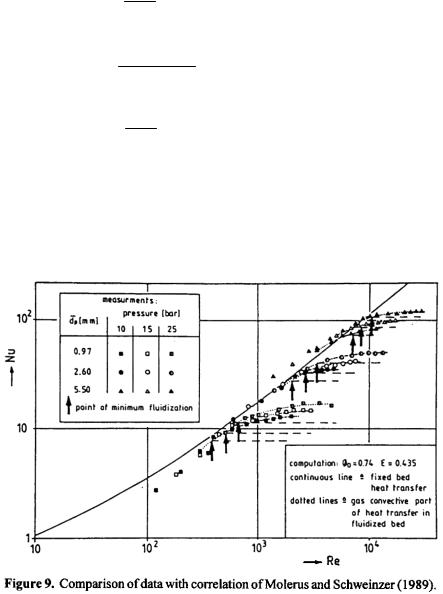
Heat Transfer in Fluidized Beds 171
The analyses reviewed above were concerned with the contribution due to dense-particle phase conduction/convection. As noted earlier, experiential measurements do indicate that the total heat transfer coefficient increases with increasing temperature. Some significant portion of this increase may be attributed to changes in the physical properties of the gas and solid phases with temperature, resulting in altered hydrodynamics and increasing the dense phase heat transfer coefficient (hd). There are contradictory opinions regarding the significance of radiative heat transfer. Ozkaynak et al. (1983) summarized the results from eight different investigations and found significant disagreement regarding the importance of radiative heat transfer. For fluidized beds of approximately the same size particles at a common bed temperature of 1,000oC, the radiative contribution to total heat transfer reported by three different investigations varied from 2% to 21%. Ozkaynak’s experiments showed that at bed temperatures below 500oC, the radiant contribution to total heat transfer is less than 15%. Radiant contribution increases linearly with bed temperature, becoming greater than 35% when bed temperature exceeds 800oC. The fact that this increase was not more rapid, i.e., proportional to fourth power of absolute temperature, may be attributed to the fact that convective/ conductive heat transfer also increased with temperature due to changes in physical properties of the gas.
The models proposed to represent radiation transport process can be grouped into two classes. The first and simpler approach is to use some form of the Stefan-Boltzmann equation for radiant exchange between opaque gray bodies,
qr |
= |
|
|
|
σ (Tb4 |
-T w4 ) |
|
|
|
||||
|
|
1 |
- |
ö |
|
|
æ |
1- |
ö |
||||
|
æ |
|
1 |
||||||||||
Eq. (11) |
|
Aw |
ç |
|
|
eb |
÷ |
+ |
+ç |
|
ew |
÷ |
|
|
|
Ab è |
|
eb ø |
|
F |
è |
ew ø |
where F is the view factor from wall to bed
The investigation of Ozkaynak et al. (1983), with direct experimental measurements of radiant heat flux, provides indications of the magnitude and temperature dependence of the effective bed emissivity. Taking ew and F both to be unity, these investigators calculated that the effective bed emissivity for particles like sand is in the range of 0.8 to 1.0 at bed temperatures where radiation might be significant (greater than 700oC) and is fairly insensitive to the superficial gas velocity. Thus, a very simple, approximate model for radiant heat transfer in bubbling fluidized beds would be Eq. (11), with Ab = Aw , F = 1, ew = 1, and ε w approximately equal to 0.9.
172 Fluidization, Solids Handling, and Processing
The second type of model takes a more physical representation of the radiant transport process. Generally, these models recognize that radiant photons are emitted, absorbed, and scattered by the solid particles in the fluidized bed. Bhattacharya and Harrison (1976) utilized this approach to modify the alternating layer model of Vedamurthy and Sastri (1974) by permitting radiation exchange from one layer of particles with 25 neighboring layers. The emulsion particles were treated as an absorbing and emitting medium so that radiation was attenuated exponentially with distance. A more rigorous model was presented by Chen and Chen (1981) whereby the Mickley-Fairbanks packet model was modified to include simultaneous radiative and conductive heat transfer during alternating contact of the heat transfer surface with gas bubbles and particle packets. The gas phase was taken to be transparent to thermal radiation while the particle packet was treated as a radiatively participative medium with absorption and scattering. During bubble contact, radiation was directly exchanged between parallel surfaces representing the heat transfer wall and the boundary of the bubble. During packet contact, Hamaker’s twoflux formulation of radiant transport was used to describe the absorption, scattering, and emission process within the packet
|
dI |
= − (A + S)I + SJ + Aσ T4 |
|
|
dy |
|
|
Eq. (12) |
dJ |
= (A + S)J − SI − Aσ T 4 |
|
|
|||
|
dy |
|
|
where |
I, J |
= |
forward and backward radiant fluxes, respectively |
|
A |
= |
volumetric absorption coefficient |
|
S |
= |
volumetric scattering coefficient |
The one dimensional transient energy equation completed the system of equations.
Eq. (13) |
k pa |
∂2T |
+ A(I + J )− 2 Aσ T 4 = ρ s C ps |
(1 − ε s, pa ) |
∂T |
|
∂y 2 |
∂t |
|||||
|
|
|
These authors numerically solved the system of equations with appropriate boundary conditions to derive the time-averaged radiant and conductive heat fluxes between the fluidized bed and the heat transfer surface. Using
Heat Transfer in Fluidized Beds 173
approximate estimates for the radiant absorption and scattering coefficients, as well as for the packet residence times, this model was shown to agree with the experimental data of Baskakov et al. (1973) Brewster and Tien (1982) made a significant contribution to the solution of this problem by showing that particles act as independent point scatters as long as the interparticle clearance is greater than 1/3 wave lengths. The implication is that almost all packed or fluidized beds can be approximated as media of independent scatterers, thereby permitting direct estimation of the volumetric scattering coefficient from properties of individual particles. Cimini and Chen (1987) used a modulated flux technique to measure radiant transmission through both packed and fluidized beds of different size glass spheres. Their data showed that the independent scattering approximation gave a reasonable, though slightly low, prediction of the radiation transmission.
3.0CIRCULATING FAST FLUIDIZATION
3.1Hydrodynamic Characteristics
As noted earlier, increasing gas velocity for any given fluidized bed beyond the terminal velocity of bed particles leads to upward entrainment of particles out of the bed. To maintain solid concentration in the fluidized bed, an equal flux of solid particles must be injected at the bottom of the bed as makeup. Operation in this regime, with balanced injection of particles into the bed and entrainment of particles out of the bed, may be termed “fast fluidization,” FFB. Figure 10 presents an approximate map of this fast fluidization regime, in terms of a dimensionless gas velocity and dimensionless particle diameter.
é |
|
ρ g2 |
ù |
|
u * = u ê |
|
|
|
ú |
μ g ( |
ρ s - ρ g |
|
||
ê |
)g ú |
|||
ë |
|
|
û |
1 / 3
Eq. (14) |
é |
ρ g (ρ s - ρ g )g |
ù |
1 / 3 |
|
d p* = d p ê |
|
ú |
= Ar 1 / 3 |
|
2 |
|||
|
ê |
ú |
|
|
|
μ g |
|
||
|
ë |
û |
|
