
Invitation to a Contemporary Physics (2004)
.pdf
9.2. Fundamental Forces |
335 |
Fortunately, things are not so bleak! We could have said the same thing about the classification of matter, for certainly there is a great variety there also. But we now know that all bulk matter is made up of electrons and quarks. Similarly, it turns out that all these complicated forces are nothing but very complex manifestations of four fundamental forces! In order of decreasing strength, these are: the nuclear force, the electromagnetic force, the weak force, and the gravitational force.
Before we go into the details of these four fundamental forces, it might be interesting to reflect on what kind of a world we would live in should these forces be very di erent from what they are.
If the electromagnetic force holding the electrons and the nucleus together had a short range and was very weak, then there could be no atoms and no molecules, because electrons could no longer be bound to the nuclei. The whole world would be a soup of negatively charged electrons and positively charged nuclei, in what is known as a plasma. Needless to say we would no longer be present. Now think of the other extreme: if the electric force were much stronger than the nuclear force. Then, the electrostatic repulsion between the protons in the nucleus would overcome the nuclear attractive forces between them, and a nucleus containing more than one proton would be broken up. The world would be left with only one chemical element, hydrogen, though hydrogen isotopes could exist and it is conceivable that very large molecules of hydrogen could be formed. In any case this world would also look very di erent from ours.
At both these extremes, when the electromagnetic force is very weak or very strong, it is almost impossible to see anything. In the former case, when the world is a plasma, light is constantly emitted and absorbed by the charged particles in the plasma. This means that light can never travel very far, and we cannot really see clearly like we do in an electrically neutral surrounding when this does not happen. At the other extreme, when the electromagnetic force is very strong, ironically we cannot see either. The world is now neutral, but another e ect takes over to block our vision. In the present world, light can travel great distances as long as there is no matter blocking its way. It is of no importance whether there are other light beams traveling in di erent directions crossing our light beams — they just go through each other. There are no tra c police in the sky, no tra c lights, but there are never collisions between the light beams in the sky. (Otherwise whatever image light brings would be all shattered by the collisions from cross beams and you would never be able to see anything.) Alas, this happy phenomenon would no longer be a reality if the electromagnetic force were very strong. In that case, it would be easy for the light beam to create ‘virtually’ an electron-positron pair, which is charged and can thus absorb and emit light. In this way an e ective plasma would be present in the vacuum, which as we have seen, would severely limit our vision. The di erence between this ‘virtual’ plasma and the real one of the previous case, is that none of the pairs in this virtual plasma can live very long. But as one dies, others are born, and there are always some pairs there to obstruct the passage of the light beam.

336 |
Elementary Particles and Forces |
If the nuclear force holding the nucleons together were too weak, we would not have complex nuclei. On the other hand, if the nuclear force were very much stronger than it is, nuclei could be much larger and very heavy chemical elements could be formed. We can go on and on with di erent scenarios. If the gravity were too weak? Then, we would all float away like an astronaut in orbit.
So the kind of world we have around us depends very sensitively on what forces are present and how strong they are. Our mere existence depends on that too. It is therefore intellectually important to understand the origin and the detailed nature of the fundamental forces. We will indeed discuss this very important and fascinating problem, but in later sections. For now, let us first get familiar with these forces.
It is ironic that the first of these four fundamental forces to be discovered is actually the weakest in strength. It is the gravitational force. This discovery was made by Isaac Newton in the seventeenth century while endeavoring to explain Kepler’s three laws of planetary motion. He found out that the planetary motions could be explained by postulating a universal attractive force between any two bodies. The force has to be proportional to the product of the masses of the two objects, and inversely proportional to the square of the distance separating them, no matter what the distance is. For this reason this force law is also known as an inverse-square law. This force is universal and all pervasive. It exists between any two of us, but we do not feel a force pulling us towards the other person because neither of us is massive enough. In contrast, the earth is heavy and it is this force that holds us to the surface of the earth. Similarly, it is this force that keeps the planets going around the sun, and the satellites going around the earth, like a weight at the end of a string. Without this force they would fly apart in a straight line. This force also exists between two elementary particles, but it is so feeble compared to the rest of the forces present that it can be safely neglected in present-day experiments. However, it may play a very fundamental role in some future theories. We will come back to this aspect in the last section.
Newton’s law of gravitation was modified and improved by Albert Einstein in his famous 1915 theory of general relativity. The result di ers from Newton’s law in mathematical details, but this di erence produces only very small e ects on planetary motions and day-to-day gravitational actions. If you should fall o a ladder, God forbid, Einstein would neither help you nor hurt you any more than Newton.
This is not to say that Einstein’s general relativity is trivial or insignificant. On the contrary, it is probably the deepest theory in the 20th century. It turns gravity into a geometrical concept, by interpreting gravity as a revelation of the curvature of spacetime. As such, light must fall under gravity just like particles do, because both of them are subjected to the same curvature. Observations show that light passing near a massive body is indeed pulled towards that body by gravity, just the way Einstein predicted. This property is nowadays used to find dark objects and dark matter in the universe. In fact, wherever there is energy, there is gravity, according

9.2. Fundamental Forces |
337 |
to Einstein. From that point of view, light is a ected by gravity because it carries energy. The faster a particle is trying to run away from the capture of gravity, the larger is the force so that eventually (this occurs only at unbelievably high energies) it does not win and cannot get away. According to Einstein, gravitational waves can be emitted from an accelerating object just like an accelerating charge can emit electromagnetic waves. This phenomenon has actually been seen in a binary pulsar (see Chapter 8) whose orbit decays because of the loss of energy due to gravitational wave emission.
Quantum gravity is not well understood so we will not discuss it much further. Su ce it to say that it is an important problem to solve, because much of our eventual understanding of particle physics may hinge on our understanding of quantum gravity.
The next fundamental force to be discovered was the electromagnetic force. Electricity and magnetism were first thought to be two unrelated forces, but subsequent experimentation showed that they were intimately connected, a connection that was finally codified and formulated into the famous Maxwell’s equations by James Clerk Maxwell in 1873. Under ordinary circumstances, the electric force is stronger than the magnetic force. Like gravity, the electric force also obeys an inverse-square law, called Coulomb’s law. The di erence is, instead of being proportional to the masses of the two bodies, the electric force is proportional to the product of their electric charges. Since electric charges can be both positive and negative, electric forces can be attractive (between opposite charges) or repulsive (between charges of the same sign). In contrast, masses are always positive and the gravitational forces are always attractive. Antigravity does not exist; even antiparticles have positive masses.
It is this bipolar property of the electric force that allowed the gravitational force to be discovered. Because of this property, there is no electric force between neutral objects, for the electric force on (or from) the positively charged part of the object is canceled by the electric force on (or from) the negatively charged part of the object. If this were not the case, the stronger electric force between the planets and the sun would have masked completely the gravitational force and prevented its discovery by Newton.
The alert reader may have noticed a peculiar feature of these two forces. The gravitational forces are attractive between masses of the same sign (because masses are necessarily non-negative), while the electrical forces are repulsive between charges of the same sign. The di erence comes about because of the di erent nature of these two forces, in spite of the fact that both of them obey the inverse-square law. We will come back to this point later in the next section.
The electromagnetic force is the force responsible for the creation of light, radio, and television waves. It is also responsible for the functioning of electronics. It is the force that binds electrons to the nucleus to make an atom, the atoms together to make a molecule, and the molecules or atoms together to form a liquid or a solid. It is the fundamental force, when dressed up in a complicated way by the presence

338 Elementary Particles and Forces
of these atoms and molecules, which gives rise to the strength of a piece of rope, or the adhesion of crazy glue. In short, other than gravity, the electromagnetic force is the only force we encounter in our daily lives.
The remaining two fundamental forces were discovered in the twentieth century. They are the nuclear or strong force, and the weak force. They are not detectable in our daily lives because of their incredibly short ranges. Nuclear forces between two nucleons are e ective only when they are within 10−15 m (one million-billionth of a meter) of each other. Weak forces have a shorter range still: about 10−17 to 10−18 m. At distances short compared to their ranges, these two forces also obey the inverse-square law. Beyond these distances, as is the case in our daily lives, these forces can become extremely small.
The great strength of the nuclear force can be seen from the fact that an enormous amount of energy can be derived from a small quantity of fissionable material used in a nuclear power plant or an atomic bomb.
The weak force is responsible for the instability of neutrons, when left alone. Under the influence of this force, an isolated neutron is torn into a proton, an electron, an anti-electron-neutrino (n → p + e + ν¯e) within a time of some fifteen minutes or so, in a process known as radioactive beta decay, (or β-decay). Under the influence of the strong or the electromagnetic force, some hadrons also become unstable, but their lifetimes are typically many orders of magnitude shorter than the neutron lifetime. This shows that the strength of the force responsible for beta decay is weak compared to the other two—hence the name ‘weak force.’
Neutrons in a nucleus are protected by the nuclear and the electromagnetic forces, so they may remain stable if there are not too many of them. If there are too many, then such protection would not be su cient for all of them to remain secure, and the nucleus would undergo a radioactive beta decay. If you have seen a watch with glowing dials, you have probably seen beta decay at work. A radioactive material is painted on the dial, whose emitted electron excites the nearby fluorescent material to produce light.
As a result of the beta decay, a neutron inside the nucleus is changed into a proton, while the produced electron and the anti-electron-neutrino leave the nucleus. The new nucleus has one more proton than the old nucleus, and soon it will capture an electron somewhere to neutralize it. Since the chemical nature of an element is determined by the number of electrons or protons, a chemical element undergoing a beta decay changes into another chemical element. With one more proton, the additional electrostatic repulsion causes the new nucleus to have a higher energy, so β-decay of the original nucleus can take place only if the energy released from the neutron β decay, about 0.8 MeV, is su cient to supply this energy di erence. Otherwise the original nucleus will remain stable in spite of the tendency of its neutron to decay.
When such a chemical change was discovered by Rutherford at the beginning of the 20th century, it amounted to heresy, especially among the chemists. It seemed

9.3. Theory of Forces |
339 |
like modern alchemy; it certainly violated the cherished ‘unalterable and indestructible’ doctrine of Dalton’s atomic theory. For this reason when Rutherford gave a talk on this discovery at the Physical Society of McGill, he was severely attacked and ridiculed by his chemistry colleague Frederick Soddy. Eventually Soddy became a convert and a close collaborator of Rutherford, and both Rutherford and Soddy went on to win separate Nobel Prizes in chemistry (1908 and 1921 respectively).
The strong force is the strongest of the four forces. As remarked above, the force between two hadrons has a range of the order of 10−15 m. The force between quarks, however, appears to be quite peculiar. At short distances, the force obeys the inverse-square law like everything else. From a distance of the order of 10−15 m onwards, the force is constant, and quite independent of the separation of the two quarks. That means no matter how far apart these two quarks are, there is always a constant force to pull them back. It also means that the work done and the energy required to pull the two quarks apart is proportional to their separation, hence an infinite amount of energy is needed to separate and isolate them. As a result, two quarks cannot be isolated from each other, a property that has come to be known as confinement. Note that hadrons such as nucleons are made out of quarks, and there is no doubt that hadrons can be isolated. So a group of quarks is sometimes confined, sometimes not. We will come back in Sec. 9.5 to discuss which is which. We should also note here that the idea of quark confinement is actually forced upon us by the failure to find isolated quarks in spite of an intense search.
9.2.1Summary
All forces are complex manifestations of four fundamental forces. In order of decreasing strength, these are the nuclear (or strong) force, the electromagnetic force, the weak force, and the gravitational force. The range of the gravitational and electromagnetic forces is long, and the range of the nuclear and weak forces is short.
9.3 Theory of Forces
We saw in the previous section that our mere existence, as well as the character of the whole universe, depend critically on the range, strength, and various other details of the fundamental forces. The electromagnetic and gravitational forces have long ranges and they obey the inverse-square law, but the weak and the nuclear forces have only short ranges. What makes them di erent, and why are some of the forces attractive and others repulsive?
These questions are unanswerable in classical physics, nor in non-relativistic quantum mechanics of atoms and molecules. A force is just there. One can measure it to determine what it is, and one can ask how it a ects the motion of particles. But one has absolutely no idea where it comes from, and why it is in a particular form.
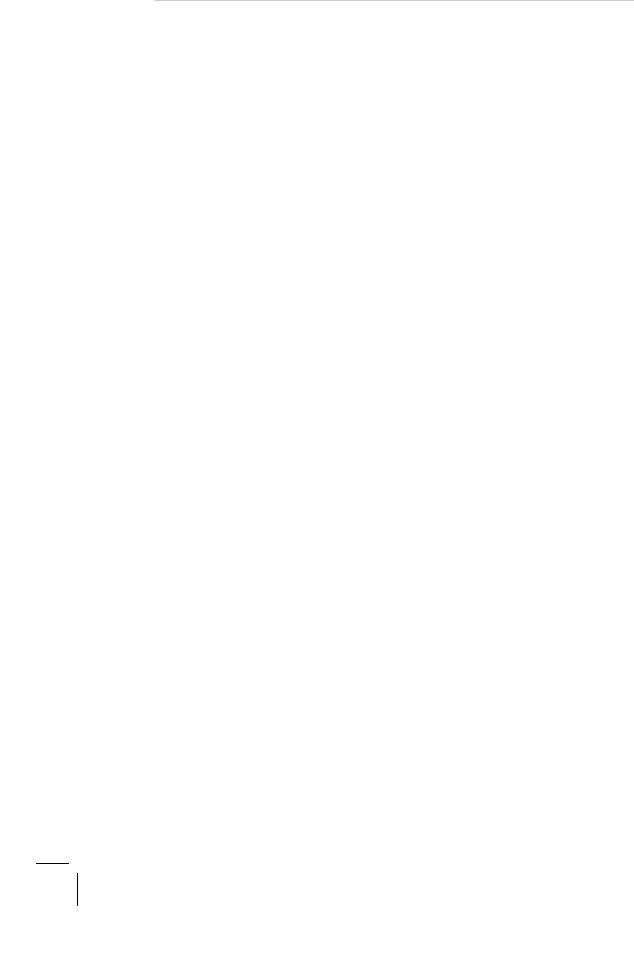
340 Elementary Particles and Forces
However, when special relativity is incorporated into quantum mechanics, these questions become answerable in the resulting quantum field theory. On the one hand, Einstein’s special relativity tells us that a particle with mass m has an energy E = mc2 at rest, and a larger one in motion. Here c = 3 × 108 m/s is the speed of light. If that amount of energy is made available, a particle of that mass can be created as long as no conservation law is violated. On the other hand, Heisenberg’s uncertainty relation in quantum mechanics (see Appendix B) tells us that the energy of a closed system may fluctuate by an amount ∆E /∆t during a time interval ∆t, where = 6.6 × 10−25 GeV s is Planck’s constant, and 1 GeV = 109 eV = 0.16×10−9 J is the energy gained by an electron crossing a billion (109) volts. Thus, at short time intervals, enough energies are available to create one or more particles. A particle of mass m thus created can last only a time interval ∆t /mc2 before it is absorbed or annihilated, or else the uncertainty principle is violated. For that reason such a particle is known as a virtual particle.
A virtual particle created by particle A at one location and annihilated by particle B at another transmits a force between A and B. This is how force arises in a relativistic quantum field theory. The virtual particle which transmits the force is sometimes known as the exchange particle.
9.3.1Range and Mass
Even moving at the speed of light, the virtual particle can travel only a distance ∆x c∆t /mc before it is absorbed, so the range of the force is of the orderc/mc2. This argument, invented by Hideki Yukawa in 1935, was used by him to predict the existence of a new particle, nowadays called the pion (π).
It was already known at that time that the range of the nuclear force is about 1 fm = 10−15 m. Using the value c = 0.197 GeV fm, the rest energy mc2 of the virtual particle is approximately c/1 fm = 0.197 GeV. After the discovery of the pion (whose rest energy is actually 0.14 GeV), Yukawa was awarded a Nobel Prize.
We know now that the pion is not an elementary particle, but that does not a ect its ability to transmit a force. There are actually three kinds of pions, π+,
0 − + ¯ − 0
π , π . Their quark compositions are π = ud, π = du¯, and π is a combination
¯
of uu¯ and dd.
Nuclear forces can also be transmitted by heavier mesons. They give rise to forces with a shorter range.
If there is no other particle nearby, the virtual particle created must be reabsorbed by A. Consequently every particle A is surrounded by a Yukawa cloud of virtual particles, whose extent is c/mc2. The density of the cloud depends on how often the virtual particle is created, which in turn depends on the strength of the local interaction. If the cloud is relatively dense, as will be the case in strong interactions, then the e ective size of the particle A will be c/mc2, which is of the order of 1 fm. If the cloud is thin, as is the case for all the other interactions, the

9.3. Theory of Forces |
341 |
cloud is hardly noticeable and the e ective size of particle A is not much increased by the presence of the cloud.
The virtual particle transmitting the electromagnetic force is the photon (γ), and the virtual particle transmitting the gravitational force is the graviton (G). Both are massless so the range of these two forces is infinite.
Weak forces come in two varieties, transmitted respectively by W ± and Z0. They carry the name of charge-current interactions and neutral-current interactions, respectively. The mass of W ± and Z0 are respectively 80 and 91 GeV/c2. Taking a
round number of 100 GeV/c2, the range of the weak forces comes out to be about 2 × 10−18 m.
We talked about strong forces between two nucleons, by exchanging pions and other heavier mesons. However, these particles are made out of quarks and antiquarks. How do quarks themselves interact?
They interact by exchanging gluons (g). As mentioned before, quarks and gluons are strange objects that must be confined inside hadrons. As a result, they are not free to propagate, so the Yukawa argument is no longer valid. Nevertheless, as we shall see, the force is independent of distance, so it is surely long-ranged. Since nucleons are made out of quarks, and the nuclear forces have a range of 1 fm, how can that happen? A look at the more familiar atomic world will give us the answer.
The force between neutral atoms, the so-called van der Waals force, also has a range much shorter than the range of the Coulomb forces between electrons and/or protons. This is because an electron in one atom sees both a repulsive force from the electrons of another atom, and an attractive force from the protons of the other atom. These two forces are almost equal and opposite and they tend to cancel each other, especially when the separation between the two atoms is much greater than the size of the atoms. This is why the van der Waals force has a range much shorter than the Coulomb force. The short range nuclear force is presumably also a result of cancelation between the long-range forces from the di erent quarks inside a nucleon. In fact, the cancelation must be so complete that the range of the residue force must have a range shorter than the pion-force range. In that way, the longest range nuclear force between nucleons is governed by the exchange of pions but not gluons.
9.3.2Inverse-Square Law versus Confinement
A force tapers o to zero beyond its range. What is it going to be at a distance r small compared to its range? We asserted in previous discussions that an inversesquare law holds at these short distances. Why?
At a distance well within the range of the virtual particle, the mass of the virtual particle does not matter. The virtual particle simply spreads out from its source like a ripple. At a distance r from the emitting particle A, the surface area of a sphere is 4πr2 (see Fig. 9.1a), and the exchange particle can be anywhere on this surface.
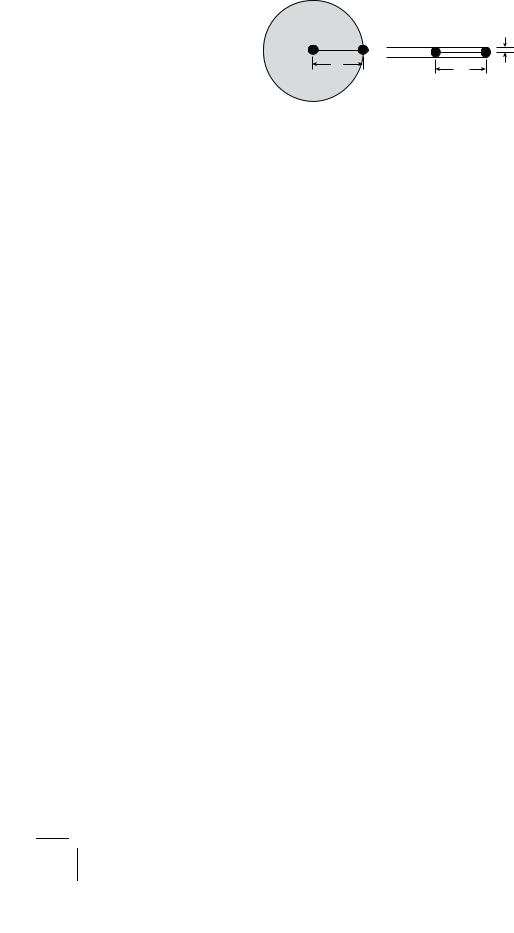
342 |
Elementary Particles and Forces |
|
r |
r |
|
|
|
(a) |
|
(b) |
Figure 9.1: (a) A sphere with radius r, illustrating the origin of the inverse-square law; (b) a cylinder of radius ρ, illustrating the origin of confinement.
The probability of its being along some fixed direction is therefore proportional to 1/r2, which is then the origin of the inverse-square law.
This argument may still be valid even if the exchange particle is not emitted isotropically. For example, if it is emitted uniformly within a 30◦ angle, then the area of the surface within this 30◦ cone would still increase like r2, and the force would still decrease like 1/r2. If the exchange particle carries a spin (see Sec. 9.3), anisotropy may indeed occur, but that will not a ect the inverse-square law at short distances for those forces.
The argument, however, fails if the emission is confined to a cylinder of constant radius ρ, as illustrated in Fig. 9.1b. For the sake of definiteness let us assume ρ R, the range of the force. At a distance r ρ, the same argument as before leads to an inverse-square law. For ρ r R, the story is di erent. The exchange particle is now guided down the cylinder, without ever having the chance to spread out into a cone. The cross-sectional area down the cylinder is constant, so the force also remains independent of r. This is what happens to the confinement force between quarks, where ρ 1 fm. What is yet to be understood is why the exchange particle is confined to the cylinder. We will return to this in Sec. 9.5.4.
9.3.3 Spin and the Nature of Forces
The spin of the exchange particle also conveys important information about the force it transmits.
Spin is the angular momentum of a particle at rest. It is an intrinsic and unchangeable attribute of the particle, just like its mass. We might think of a particle as a miniscule earth, and its spin is like the rotation of earth about its own axis. While this analogy has some merit, it is somewhat dangerous because it will yield nonsensical results if taken too far. In reality, spin is a quantum mechanical phenomenon with no classical analog.
Angular momentum is described classically by a three-dimensional vector, or equivalently, by its three components (see Appendix A). Quantum mechanically, on account of the uncertainty principle, its three components are not simultaneously measurable. The best we can do is to specify the magnitude of the vector, together

9.3. Theory of Forces |
343 |
with one of the three components. It is traditional to label the component specified to be the z-component.
The length of the spin vector is s(s + 1) , and the z-component is ms . s must
either be a non-negative integer (s = 0, 1, 2, . . .), or a half-integer (s = 12 , 32 , 52 , . . .). For a given s, ms may take on 2s+1 discrete values, from −s, −s+1, . . . , to s−1, s.
Electrons, nucleons, quarks, and neutrinos are s = 12 objects, and so are their antiparticles. Photons, gluons, W ± and Z0 are spin-1 objects, and the graviton is a spin-2 object. The Higgs boson φ0 has a spin 0.
Since the x- and the y-components cannot be fixed once the z-component is specified, we might think of the spin vector as precessing in a cone, as shown in Fig. 9.2.
The nature of the force carried by an exchange particle depends on the spin of that exchange boson.
Quantum field theory dictates that if s is an even integer, the force between identical particles is attractive. If s is an odd integer, the force between identical particles is repulsive, and the force between a particle and its own antiparticle is attractive.
Pions have spin s = 0 and gravitons have spin-2. This is the reason why the nuclear and the gravitational forces of two protons are both attractive. Photons have spin-1, so the Coulomb force between two protons is repulsive.
The spin of the exchange particle also determines the complexity of the force. An exchange particle carries with it 2s + 1 bits of information on its orientation. These di erent bits can trigger di erent forces, so the number and complexity of the force increases with the value of the spin. For example, the Yukawa nuclear force between two nucleons is carried by the pion, which has spin-0. So the force is a single attractive one. The electromagnetic force between two protons, however, is
z
s m
|
S(S+1) |
|
√ |
|
|
|
|
y
x
Figure 9.2: The thick arrow represents a quantum-mechanical angular momentum vector, which can be pictured to be precessing about the z-axis on the surface of the cone.

344 |
Elementary Particles and Forces |
carried by a photon, which has spin-1. As a result, there is in this case an electric force and a magnetic force. Gravitational force is transmitted by a spin-2 graviton, so the force is even more complex than the electromagnetic one. Nevertheless, in all these cases, unless the particles move very fast, the magnetic-like force is much smaller than the electric-like force, so the latter dominates. In the case of electromagnetism, this is the Coulomb force. In the case of gravity, this is the Newtonian force. When we talked about the association between the spin of the exchange particle and the type of force it transmits, we were tacitly referring to these dominant forces.
9.3.4 Energy and Force
Many people find it di cult to understand how the exchange of a virtual particle can possibly produce an attractive force. They argue that if A emits a virtual particle towards B, then A must recoil away from B. When B absorbs this virtual particle and its momentum, B must be knocked away from A. Therefore the exchange always causes A and B to move away from each other, which is the hallmark of a repulsive force. How can one possibly get an attractive force?
This argument would have been correct in the absence of quantum mechanics, but then we would not have the virtual particle either. Since the distance between A and B are fixed, the uncertainly principle tells us that the momentum of the virtual particle is undetermined. It could be opposite to what it is classically and intuitively, thereby causing A and B to move towards each other. This gives rise to an attractive force.
How then is one going to determine whether a force is attractive or repulsive? To that end it is better to discuss energy, and not the force directly.
The interaction of A and B, separated by a distance r, is determined by a potential energy V (r). A physical system likes to minimize its potential energy by moving its particles towards a smaller V (r). The sharper the variation of V (r), the faster the particles move. The corresponding force is simply F = −dV (r)/dr.
In quantum field theories, the potential energy V (r) consists of three factors, V (r) = CAPs(r)CB. The factor Ps(r) fse /r, called the Yukawa potential, tells us how the virtual particle propagates. It is the only factor which depends on the distance r. From the exponential factor it is clear that the range is given by R /mc, as demanded by the Yukawa theory. The factor fs depends on the spin s of the exchange particle. For the dominant force, it is negative for even s and positive for odd s. This sign determines whether the corresponding force is attractive or repulsive.
The inverse-square law at short range now follows from the formula
F = −dV (r)/dr when r R .