
Molecular Sieves - Science and Technology - Vol. 6 - Characterization II / 04-NMR of Physisorbed 129Xe Used as a Probe to Investigate Molecular Sieves
.pdf
Mol Sieves (2007) 5: 155–248 DOI 10.1007/3829_004
♥ Springer-Verlag Berlin Heidelberg 2006 Published online: 10 February 2006
NMR of Physisorbed 129Xe Used as a Probe to Investigate Molecular Sieves
J.-L. Bonardet1 · A. Gédéon1 · M.-A. Springuel-Huet1 · J. Fraissard2 ( )
1Laboratoire S.I.E.N., University P. and M. Curie, 4 place Jussieu, 75252 Paris Cedex 05, France
2Laboratoire PMMH, University P. and M. Curie, ESPCI, 10 rue Vauquelin, 75231 Paris Cedex 05, France
jfr@ccr.jussieu.fr
1 |
General Introduction . . . . . . . . . . . . . . . . . . . . . . . . . . . . . |
158 |
1.1 |
Introduction . . . . . . . . . . . . . . . . . . . . . . . . . . . . . . . . . . |
158 |
1.2 |
NMR Properties of Xenon . . . . . . . . . . . . . . . . . . . . . . . . . . . |
159 |
1.3 |
129Xe-NMR Chemical Shift in Xenon Gas . . . . . . . . . . . . . . . . . . |
162 |
1.4 |
129Xe NMR Chemical Shift in Mixtures of Xenon and Other Gases . . . . |
163 |
2 |
Zeolites . . . . . . . . . . . . . . . . . . . . . . . . . . . . . . . . . . . . . |
164 |
2.1 |
129Xe-NMR of Adsorbed Xenon: Generalities . . . . . . . . . . . . . . . . |
164 |
2.2 |
Models for the Interpretation of Chemical Shifts Due to the Porosity . . . |
166 |
2.2.1 |
Introduction . . . . . . . . . . . . . . . . . . . . . . . . . . . . . . . . . . |
166 |
2.2.2 |
Fast Site Exchange . . . . . . . . . . . . . . . . . . . . . . . . . . . . . . . |
166 |
2.2.3 |
Influence of Surface Curvature . . . . . . . . . . . . . . . . . . . . . . . . |
168 |
2.2.4 |
Lennard-Jones Potential Curves . . . . . . . . . . . . . . . . . . . . . . . . |
170 |
2.2.5 |
Discussion . . . . . . . . . . . . . . . . . . . . . . . . . . . . . . . . . . . |
172 |
2.2.6 |
Porosity Studies . . . . . . . . . . . . . . . . . . . . . . . . . . . . . . . . |
175 |
2.3 |
Influence of Cations . . . . . . . . . . . . . . . . . . . . . . . . . . . . . . |
183 |
2.3.1 |
Introduction . . . . . . . . . . . . . . . . . . . . . . . . . . . . . . . . . . |
183 |
2.3.2 |
Case of H and Alkali-Metal Ions . . . . . . . . . . . . . . . . . . . . . . . |
183 |
2.3.3 |
Influence of Divalent Cations: Diamagnetic and Paramagnetic Ions . . . . |
184 |
2.3.4 |
Case of Trivalent Cations . . . . . . . . . . . . . . . . . . . . . . . . . . . |
186 |
2.3.5Effect of the Electronic Structure (nd10):
|
Ag+, Cu+, Zn2+, and Cd2+ Cations . . . . . . . . . . . . . . . . . . . . . . |
187 |
2.3.6 |
129Xe NMR . . . . . . . . . . . . . . . . . . . . . . . . . . . . . . . . . . . |
189 |
2.3.7 |
Cation Exchange Between Different Zeolites . . . . . . . . . . . . . . . . . |
191 |
2.3.8 |
Effect of Nonframework Aluminum . . . . . . . . . . . . . . . . . . . . . . |
193 |
2.4 |
Bulk and Distribution of Adsorbed Phases . . . . . . . . . . . . . . . . . . |
194 |
2.4.1 |
Introduction . . . . . . . . . . . . . . . . . . . . . . . . . . . . . . . . . . |
194 |
2.4.2 |
Study of Adsorbed Organic Molecules . . . . . . . . . . . . . . . . . . . . |
194 |
2.4.3 |
Study of the H2 O/Na–Y System . . . . . . . . . . . . . . . . . . . . . . . . |
198 |
2.4.4 |
Study of Coke Deposition in Zeolites . . . . . . . . . . . . . . . . . . . . . |
199 |
2.5 |
Study of Supported Metals and Chemisorption Thereon . . . . . . . . . . |
203 |
2.5.1 |
Introduction . . . . . . . . . . . . . . . . . . . . . . . . . . . . . . . . . . |
203 |
2.5.2 |
Chemisorption of Hydrogen . . . . . . . . . . . . . . . . . . . . . . . . . . |
205 |
2.5.3 |
Chemisorption of other Gases (G) . . . . . . . . . . . . . . . . . . . . . . |
209 |
2.5.4 |
Metals other than Platinum . . . . . . . . . . . . . . . . . . . . . . . . . . |
210 |
156 |
|
J.-L. Bonardet et al. |
2.6 |
Xenon Diffusion . . . . . . . . . . . . . . . . . . . . . . . . . |
. . . . . . . 210 |
2.6.1 |
Introduction . . . . . . . . . . . . . . . . . . . . . . . . . . . |
. . . . . . . 210 |
2.6.2 |
Self-Diffusion of Xenon by the PFG NMR Technique . . . . . |
. . . . . . . 210 |
2.6.3 |
Dynamics of Adsorbed Xenon and 129Xe Chemical Shift . . . |
. . . . . . . 214 |
2.6.4129Xe NMR as a Tool for Probing Intracrystalline Concentration Profiles
|
and Transport Diffusion . . . . . . . . . . . . . . . . . . . . . . . . . . . . |
221 |
3 |
Other Microporous Solids . . . . . . . . . . . . . . . . . . . . . . . . . . . |
223 |
3.1 |
Pillared Clays . . . . . . . . . . . . . . . . . . . . . . . . . . . . . . . . . . |
223 |
3.2 |
Heteropolyoxometalate Salts (HPOM) . . . . . . . . . . . . . . . . . . . . |
225 |
3.3 |
Activated Carbon . . . . . . . . . . . . . . . . . . . . . . . . . . . . . . . . |
227 |
4 |
Mesoporous Solids . . . . . . . . . . . . . . . . . . . . . . . . . . . . . . . |
228 |
4.1 |
Amorphous Oxides . . . . . . . . . . . . . . . . . . . . . . . . . . . . . . . |
228 |
4.2 |
New Mesoporous Solids (MCM, SBA, etc.) . . . . . . . . . . . . . . . . . . |
234 |
4.3 |
Xe NMR Cryoporometry . . . . . . . . . . . . . . . . . . . . . . . . . . . . |
235 |
5 |
Conclusions . . . . . . . . . . . . . . . . . . . . . . . . . . . . . . . . . . . |
237 |
6 |
Appendix: Hyperpolarized Xenon . . . . . . . . . . . . . . . . . . . . . . |
238 |
References . . . . . . . . . . . . . . . . . . . . . . . . . . . . . . . . . . . . . . . |
240 |
Abstract Xenon is an inert gas with a very large electron environment that makes it sensitive to any interaction, even physical. In the case of 129Xe isotope (spin 1/2), the resulting electronic perturbation is directly transmitted to the nucleus and, therefore, affects the nuclear magnetic resonance chemical shift. In this chapter, we report some applications of this technique in both fundamental and applied research in the field of microporous and mesoporous solids.
Keywords 129Xe-NMR · Optically polarized xenon · Zeolites · Microporous solids · Mesoporous solids · Clays · Carbon · Adsorption · Porosity · Metal catalysts
Abbreviations |
|
aXe |
Xe atom radius (0.22 nm) |
A |
internal surface area |
An |
preexponential factor of kn |
b |
ka /kd ratio |
C |
molecular constant characterizing the polarizability (Sect. 2.2.3), relative |
|
water concentration in zeolite (Sect. 2.4.3) |
d |
shortest distance from Xe to the shell wall in potential calculation |
|
(Sect. 2.2) |
D |
Xe atom diameter (0.44 nm), diffusion coefficient (Sect. 2.6) |
D0 |
pre-exponential factor of diffusion coefficient |
Ea (n) |
activation energy relative to kn |
g |
magnetic-field gradient in pulsed-field gradient NMR |
Ia , Ib, Ic |
intensity of line a, b, c, respectively |
k |
Boltzmann constant |
ka |
rate constant of adsorption |
NMR of Physisorbed 120XE Used as a Probe to Investigate Molecular Sieves |
157 |
|||
kd |
|
rate constant of desorption |
|
|
kn |
|
rate coefficient for a given Xe atom to leave a n-cage |
|
|
|
|
|
mean free path of a Xe atom adsorbed in a microporous solid |
|
L |
|
pore width (Sect. 2.2.4) |
|
|
M(ω(n), ω(m)) |
joint probability of finding a Xe atom in a n-cage before tmix and in a m- |
|||
|
|
|
cage afterwards |
|
n-cage |
Na – A cavity containing n (m) atoms |
|
||
na |
|
number of adsorption sites in a unit volume |
|
|
nEM |
number of metal particles determined by electronic microscopy |
|
||
nH2 |
number of chemisorbed H2 molecules |
|
||
np |
|
number of metal particles |
|
|
N |
|
number of adsorbed Xe atoms per gram of dehydrated solid |
|
|
NA |
|
number of Xe atoms located on surface sites of the internal pore wall |
|
|
NV |
number of Xe atoms situated in the internal pore volume |
|
||
NS |
|
number of adsorbed Xe per cavity |
|
|
tmix |
mixing time in 2D NMR experiment |
|
||
T |
|
absolute temperature (Kelvin) |
|
|
p |
|
probability of a xenon atom being at the cavity wall (Sect. 2.2), number of |
||
|
|
|
differentiated particles (Sect. 4) |
|
P |
|
gaseous pressure |
|
|
P(Xe) |
equilibrium Xe pressure |
|
||
P(n) |
probabilty of finding a n-cage among all of them |
|
||
2 |
(∆) intra |
mean square displacement of molecules diffusing inside the crystallites |
||
r2 |
||||
r |
(∆) inter |
mean square displacement of molecules diffusing outside the crystallites |
||
R |
|
radius of the spherical shell representing the cage wall (Sect. 2.2.4), radius |
||
|
|
|
of adsorbent particle or gas constant |
|
Rn,m |
overall rate of Xe atoms going from a n-cage to a m-cage |
|
||
Si,j. . . |
surface of an i(j. . .)-type particle |
|
||
Vi,j. . . |
part of the volume of zeolite containing i(j. . .)-type particles |
|
||
W |
|
van der Waals interaction energy (Sect. 2.2.3), concentration of Na – Y or |
||
|
|
|
ZSM-5 zeolite in a mixture with Na – A (Sect. 2.6.3) |
|
αi |
|
probability of Xe – Si collision |
|
|
αNa–Y |
probability of Xe – Na – Y zeolite wall collision |
|
||
γ |
|
gyromagnetic ratio |
|
|
γ (∆) |
relative amount of molecules diffusing in intercrystallite space |
|
||
∆H |
NMR linewidth |
|
||
δw |
|
solvent effects on chemical shift due to van der Waals interactions |
|
|
δa |
|
chemical shift of a Xe atom located on surface sites of the internal pore |
||
|
|
|
wall, chemical shift of line a (Sect. 2.2) |
|
δb, δc |
chemical shift of line b and c, respectively (Sect. 2.5.2) |
|
||
δi |
|
chemical shift of Xe atoms interacting with an i-type particle |
|
|
δNa–Y |
chemical shift of Xe atoms interacting with the Na – Y pore wall |
|
||
δV |
|
chemical shift of a Xe atom situated in the internal pore volume |
|
|
δV |
average value of δV |
|
||
δS |
|
chemical shift of adsorbed Xe due solely to interactions with the internal |
||
|
|
|
pore wall, δS is a weighted average of δa and δV |
|
δXe |
contribution of Xe – Xe interactions to the chemical shift |
|
||
δSAS |
contribution of Xe-strong adsorption site interactions to the chemical shift |
|||
δE |
|
contribution of electric field due to the cations to the chemical shift |
|
|
δM |
|
contribution of cation paramagnetism to the chemical shift |
|
158 |
J.-L. Bonardet et al. |
δN →0 |
chemical shift extrapolated to zero concentration |
δ0 |
chemical shift of an isolated Xe atom |
δref |
same meaning as δ0 |
δ1 , δ2, δ3 |
characterize the Xe – Xe interactions in the virial expansion of the xenon |
δ |
density |
pulse width in pulsed-field gradient NMR |
|
∆ |
time separation between two identical field-gradient pulses in pulsed-field |
|
gradient NMR |
ε |
energy coefficient in Lennard-Jones potential function |
λ |
fitting parameter in δS empirical expression (Sect. 2.6), degree of cationic |
|
exchange in faujasite (Sect. 3.3) |
λi |
probability of Xe collisions with i-type particles in a volume Vi |
µ |
fitting parameter in δS empirical expression |
µi |
probability of Xe collisions with Na – Y in a volume Vi |
θ |
average number of benzene molecules per cage |
ρ |
xenon density |
ρXe |
xenon density in binary mixture |
ρA |
A-gas density in binary mixture (Xe-A) |
ρa |
xenon density of atoms located on surface sites of the internal pore wall |
ρV |
xenon density of atoms situated in the internal pore volume |
ρg |
concentration of xenon located in the free volume of faujasite supercages, |
|
similar to ρV (Cheung model) |
σd |
diamagnetic contribution to the shielding constant |
σp |
paramagnetic contribution to the shielding constant |
τintra |
intracrystallite mean lifetime |
τ diff |
minimum intracrystallite mean lifetime |
intra |
analogous to δ1 in the Cheung model |
ξg |
|
ψ |
intensity of the spin-echo signal in pulsed-field gradient NMR |
1
General Introduction
1.1 Introduction
The central idea of the pioneers [1] of this technique was to find a molecule which was nonreactive and particularly sensitive to its environment, to physical interactions with other chemical species and to the nature of adsorption sites, and which could, therefore, be used as a probe to determine in a new way solid properties difficult to detect by classical physicochemical techniques. In addition, this probe needed to be detectable by NMR, since this technique is particularly suitable for investigating electron perturbations in rapidly moving molecules.
The isotope 129 Xe, which the authors proposed, is this ideal probe. It has given rise in the last 25 years to a great number of applications both in fundamental and applied research. Fragmentary reviews have already
NMR of Physisorbed 120XE Used as a Probe to Investigate Molecular Sieves |
159 |
been published [2–7]. We present here some examples of the applications of the Xe-NMR technique to the study of microporous and mesoporous materials.
1.2
NMR Properties of Xenon
Xenon was discovered by Ramsay and Travers in 1898. The element has a Z value of 54. Among the nine stable xenon isotopes, only two, 129 Xe and 131 Xe, have nonzero spin and are therefore of interest for NMR studies. Table 1 gives their natural abundance, their magnetic properties [8–10] and their sensitivity to detection (Table 1).
At a field of 2.3488 Tesla, the resonance frequencies of 1H, 13C and 129 Xe are 100, 25.144 and 27.600 MHz, respectively. The resonance of 129 Xe occurs, therefore, at a frequency about 10% higher than that of 13C at the same magnetic field strength. This makes it possible to use a spectrometer designed for 13C detection with a slight change of the circuit tuning. According to the natural abundance of the various isotopes, the sensitivity of detection of 129 Xe (the isotope most widely studied) with respect to 1H and 13C
13 |
× |
10–3 and 31.8, respectively. Thus, |
129 Xe is easier to observe than |
is 5.60 |
|
C, neglecting differences in relaxation times. Of course, pure monoatomic xenon is expected to have a long spin relaxation time T1, but relaxation is
enhanced by interaction with paramagnetic species, even with few impurities. For example, 129 Xe is easily detectable in xenon gas at 0.5 MPa and at 27 MHz if 1% of O2 is added. We will see later that the T1 of 129 Xe ad-
sorbed in microporous solids is rather short, typically in the range of 10 ms to a few seconds, even at low temperature. By using optical polarization techniques (see Sect. 6) [11–13] the sensitivity of detection can be increased by several orders of magnitude, which widens the field of applications of this technique. The use of a continuous flow approach [13–17] for the production of hyperpolarized xenon (denoted HP Xe in this text) is particularly beneficial for several applications (porous materials [15], flames [16], microimaging [17], etc.).
Table 1 |
Natural abundance and magnetic properties of 129Xe and 131Xe |
|
|
|||
|
|
|
|
|
|
|
Isotope |
Natural |
Spin |
Magnetic |
Electric |
Sensitivity |
|
|
abundance in |
quantum |
moment |
quadrupole |
to detection |
|
|
xenon (%) |
number |
(µN ) |
moment |
|
|
|
|
|
|
(×10–28 m2) |
|
|
129 |
|
|
|
|
|
–3 |
131Xe |
26.4 |
1/2 |
– 0.77247 |
– 0.12 |
5.60 |
× 10–4 |
Xe |
21.2 |
3/2 |
0.68697 |
5.61 |
× 10 |
160 |
J.-L. Bonardet et al. |
In theory, the nuclear relaxation time T1 of adsorbed xenon should provide interesting information about local xenon structure and dynamics. Unfortunately, this is only true for extremely pure solids. The importance of paramagnetic impurities for relaxation is well known. Now, real catalysts, which are the most studied of all microporous solids, always contain relatively high concentrations of all sorts of impurities. For this reason we shall not discuss
relaxation very much in what follows. The most important NMR characteristic of 129 Xe is its chemical shift which ranges over 7500 ppm for all the xenon
compounds and over 1500 ppm if only adsorption is considered. This is a very wide range compared to 1H (20 ppm) and 13C (300 ppm) for diamagnetic systems. The chemistry of xenon began in 1962 [18]. Since that time many xenon compounds have been synthesized and 129Xe NMR has proved to be the best technique for determining their structure [19, 20]. General information about these xenon derivatives can be found in [21] and [22]. However, 129 Xe NMR appeared to be of general interest in many fields: in physics, to study the interactions of xenon with solvents and with organic or bioorganic ligands, etc. General information on these applications can be found in the review by Reisse [23]. As discussed by Ramsey [24] the shielding constant consists of two parts:
•a diamagnetic contribution, σd, which depends only on the fundamental state of the electronic system and can be related to the charge on the atom considered;
•a paramagnetic contribution, σp, which depends on the excited states and above all on the symmetry of the valence orbitals. The shielding constant
of the isolated xenon atom (gas pressure extrapolated to zero) is due to σd only. The paramagnetic contribution, which expresses the deviation from spherical symmetry, is zero.
In most xenon compounds the electron distribution is not spherically symmetrical and the σp values show a large variation, which depends on the nature of the atom to which the xenon is bonded, and the bond type. Except in a very small number of cases the chemical shifts, δ, measured relative to the isolated atom, are positive (downfield).
In this paper we will focus our attention on 129Xe NMR as a means of probing the properties of inorganic microporous materials such as zeolites.The very large and extremely polarizable electron cloud of xenon makes this atom particularly sensitive to its close environment. Small variations in the physical interactions with the latter cause marked perturbations of the electron cloud which are transmitted directly to the xenon nucleus and greatly affect the NMR chemical shift.
Results on liquid media amply demonstrate the sensitivity of the chemical shift of xenon to physical interactions. Figure 1 shows that 129Xe gas to solution shifts are linearly related to the 13C gas to solution shifts of methane in the same solvents. Since the shifts are attributed exclusively to van der Waals
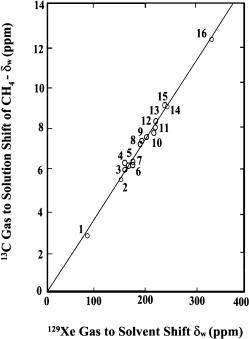
NMR of Physisorbed 120XE Used as a Probe to Investigate Molecular Sieves |
161 |
Fig. 1 Gas to solution shifts of 13C in 13CH4 in various solvents (corrected for susceptibility) vs. gas to solution shifts of 129Xe in the same solvents. 1, C6F6; 2, CH3NO2;
3, cyclo-C5 H10 ; 4, TMS; 5, cyclo-C6 H12 ; 6, CH3CN; 7, CH3COCH3; 8, C6H5NO2; 9, C6H6; 10, C6H5Cl; 11, CHCl3; 12, CCl4; 13, CS2; 14, (CH3)2SO; 15, CH3I; 16, CH2I2. (From [25], Copyright Wiley (1991). Reproduced with permission)
effects, this variation proves that 129Xe is 27.1 times more sensitive to these effects than 13C [25]. Generally speaking, in the observed solvent effects on chemical shifts the part due to van der Waals interactions, δw, is the most important for heavy atoms. δw does not depend only on the solvent (Fig. 1) but sometimes also on the nuclear site in a given solute molecule. The effect of δw on the observed chemical shifts in homogeneous media has been the subject of much theoretical work which has been very well reviewed by Rummens [26].
Finally, it should be remembered that for every chemical shift measurement with an external reference a correction of the magnetic susceptibility related to the volume should be made; this was first mentioned by Dickinson [27], then developed mainly by Bothner-By and Glick [28] for homogeneous media. Bonardet and Fraissard have described the means of performing this correction in the measurement of shifts of phases adsorbed on solids [29]. However, in the present study, given the very large shifts of adsorbed xenon, this correction is negligible.

162 |
J.-L. Bonardet et al. |
1.3
129Xe-NMR Chemical Shift in Xenon Gas
Before reviewing the NMR spectroscopy of 129Xe adsorbed in microporous structures, it seems necessary to summarize some results relative to the 129 Xe chemical shift in xenon gas or in mixtures of xenon and other gases.
After the first detection of gaseous xenon [30] the subsequent studies by Carr et al. [31–33] and by Brinkmann [34] showed that over a wide range of density the shift can be expressed quite well by a virial expansion of the xenon density ρ:
δ(T, r) = δ0 + δ1(T)ρ + δ2(T)ρ2 + δ3(T)ρ3 |
(1) |
where δ(T, ρ) is the resonance shift at a temperature T and a density ρ. δ0 is the position of the isolated atom. Experimentally this corresponds to virtually zero pressure (in what follows we shall always use this reference). δi(T) are the virial coefficients of the shift as a function of density.
The most exact values were obtained by Jameson et al. [35]. At 298.15 K:1
δ1 |
= 0.548 ± 0.004 ppm/amagat1 |
(2a) |
δ2 |
= (0.169 ± 0.02) 10–3 ppm/amagat2 |
(2b) |
δ3 |
= (– 0.163 ± 0.01) 10–5 ppm/amagat3 |
(2c) |
For densities below about 100 amagat, terms of an order higher than one are negligible. In this case, the linear variation yields a value of 0.55 ppm chemical shift per amagat. δ1 results from binary collisions. Higher-order terms are an indication that three or more body collisions become important at higher density. Various authors have calculated the value of δ1 from the interaction of two xenon atoms at the moment of collision [35, 36].
Adrian [36] has demonstrated that only the exchange interactions of the wave functions of the colliding atoms make a significant contribution to the chemical shift. In his opinion the van der Waals interactions were of negligible importance. Later, Jameson et al. showed that Adrian underestimated the role played by repulsive interactions on the pressure dependence of δ; these
interactions must be taken into account [35]. |
|
The coefficients δi in Eq. 1 are temperature-dependent |
[37, 38]. Fitting |
δ1 (T) to a polynomial of degree 4, Jameson et al. give [38]: |
|
δ1(T) = 0.536 – 0.135 × 10–2 τ + 0.132 × 10–4 τ 2 |
(3) |
– 0.598 × 10–7 τ 3 + 0.663 × 10–10 τ 4 ppm/amagat, |
|
where τ = T – 300 K. |
|
1 One amagat is the density (2.689 × 1019 atoms/cm3) of an ideal gas at standard temperature (273.15 K) and pressure (1 atmosphere).

NMR of Physisorbed 120XE Used as a Probe to Investigate Molecular Sieves |
163 |
Dybowski et al. [6] have proposed a strictly empirical function to describe the temperature dependence of δ1:
δ1(T) = 0.467 + 19.29 exp(– 0.0187T). |
(4) |
It must be remembered that the shift δ0 of an isolated xenon atom is strictly temperature-independent [38].
Finally, the paper of Cowgill and Norberg [39] shows that the effect of the xenon pressure on the chemical shift is of the same type for all three phases, gas, liquid, and solid. This means that “the repulsive exchange forces may also have a role in the liquid and solid states” [39]. This result is particularly interesting for the interpretation of the δ values of adsorbed xenon.
1.4
129Xe NMR Chemical Shift in Mixtures of Xenon and Other Gases
In a mixture of xenon with another gas A, the shift of xenon depends on the nature of A and the collision frequency with the latter. When the xenon density is so low that only terms for binary collisions need to be considered, one may generalize Eq. 1 to a multi-dimensional virial expansion of the shift [35, 38, 40]:
δ(T) = δ0 + δ1,Xe(T).ρXe + δ1,A(T).ρA, |
(5) |
where ρXe and ρA are the densities of Xe and A, respectively. Since δ1,Xe has previously been determined, δ1,A(T) can be calculated from data (Table 2).
Table 2 Measured values of second virial coefficients δ1,A (T = 298 K) of the 129Xe NMR shift for interactions with various gases A (from [35] and [40])
A |
δ1,A (ppm/mol cm–3) |
δ1,A (ppm/amagat) |
||
|
|
|
|
|
Ar |
3119 ± 216 |
0.139 |
± 10 × 10–3 |
|
CO2 |
3823 ± 151 |
0.171 |
± 7 × 10–3 |
|
CF4 |
4327 ± 121 |
0.193 |
± 5 × 10–3 |
|
CHF2 |
4287 ± 198 |
0.191 |
± 9 × 10–3 |
|
CH2F2 |
4965 ± 239 |
0.222 |
± 10 × 10–3 |
|
CH3F |
4314 ± 170 |
0.192 |
± 9 × 10–3 |
|
CH4 |
6201 ± 125 |
0.277 |
± 12 × 10–3 |
|
Kr |
6070 ± 375 |
0.271 |
± 12 × 10–3 |
|
HCl |
7678 ± 132 |
0.343 |
± 15 × 10–3 |
|
Xe |
12283 |
± 90 |
0.548 |
± 24 × 10–3 |
NO |
17769 |
± 256 |
0.793 |
± 11 × 10–3 |
O2 |
21037 |
± 216 |
0.939 |
± 10 × 10–3 |
164 |
J.-L. Bonardet et al. |
δ1,A (T) is also temperature-dependent. The effect on the xenon shift of xenonother gas interactions is smaller than that of interactions between xenon atoms, except for O2 and NO. The large shift induced by gaseous O2 and NO arises from a “contact overlap” mechanism. Overlap of the Xe (5s) and the O2 (πg ) or NO (π ) orbitals is the major contributor to the spin density at the Xe nucleus [41–43]. It should be remarked that this is the first example of a contact shift of this type.
Previously, the contact-shift was only observed when the unpaired electron was on the same molecule as the nucleus under observation. This result will also be important in the case of xenon adsorbed on paramagnetic or metallic solids.
Finally, for certain studies mentioned below, where the xenon is coadsorbed with another phase at high concentration, we felt it useful to recall the studies of Stengle et al. [44, 45] and Muller [46] concerning xenon dissolved in various hydrocarbon solvents, as well as the critical review already cited [23]. The former have shown that in the calculation of the shift of xenon, the reaction field model is valid within a group of closely related solvents, but it fails when extended to collections of randomly selected solvents. According to Muller, in calculating the van der Waals interactions one must take into account what he calls “the solvent cohesive energy density”, which corresponds to the idea of the “volume of mixing effect” [47].
2 Zeolites
2.1
129Xe-NMR of Adsorbed Xenon: Generalities
As in the gas phase the main information has been obtained from the analysis of the variation of the chemical shift against the xenon concentration, generally at 300 K. The amount of adsorbed xenon is expressed as the number, N, of atoms per gram of anhydrous zeolite or the number of atoms, Ns, per cage (zeolites Y, A, erionite, etc.).
Fraissard et al. have shown that the chemical shift of adsorbed xenon is the sum of several terms corresponding to the various perturbations it suffers [2– 4, 48].
δ = δref + δS + δXe + δSAS + δE + δM. |
(6) |
δref is the reference (gaseous xenon at zero pressure). δS arises from interactions between xenon and the surface of the zeolitic pores, provided that the solid does not contain any electrical charges. In this case it depends only on the dimensions of the cages or channels and on the ease of xenon diffusion. δXe = δXe–Xe.ρXe corresponds to Xe – Xe interactions; it increases