
Molecular Sieves - Science and Technology - Vol. 6 - Characterization II / 03-Characterization of the Pore Size of Molecular Sieves Using Molecular Probes
.pdfCharacterization of the Pore Size of Molecular Sieves |
133 |
Criterion (ii) is a quantitative expression of the observation that, with decreasing pore width, isomerization of m-xylene is more and more favored over its disproportionation. At 350 ◦C, the bimolecular disproportionation is completely suppressed in the medium-pore zeolite ZSM-5, whereas isomerization (which is likely to proceed via the monomolecular mechanism at these high temperatures) does not experience significant hindrance [85]. Later, Olson and Haag [30] demonstrated that, if zeolite catalysts with different pore systems are used, a linear relationship results between the ratio of rate constants of disproportionation and isomerization and the dimensions of the largest zeolite cavities. One of the conclusions of this study was that the suppression of disproportionation is due to restricted transition state shape selectivity rather than to mass transfer effects.
Finally, criterion (iii) was deduced from the observation that the disproportionation of m-xylene in the spacious cavities of zeolite Y and in the much narrower channels of mordenite (MOR) resulted in significantly different distributions of the trimethylbenzene isomers: Of the product obtained from H-mordenite, 94% of the trimethylbenzenes consisted of the 1,2,4-isomer. On the other hand, 74% of the 1,2,4-isomer and 24% of the 1,3,5-isomer were found in the product from H-Y. (On both zeolites, only negligible amounts of 1,2,3-trimethylbenzene were formed, and this was attributed to its low concentration in thermodynamic equilibrium [85]). To interpret the hindered formation of 1,3,5-trimethylbenzene from m-xylene in mordenite, the authors mainly invoked restricted transition state shape selectivity.
m-Xylene isomerization as a catalytic test reaction was later adopted by a number of other groups [2, 67], and the criteria proposed by Gnep et al. [85] were refined and applied to a broader structural variety of molecular sieves. Dewing [86] introduced the so-called R value, defined as the ratio of rate constants observed experimentally for the formation of o- and p-xylene from m-xylene under conditions of diffusional limitations. Joensen et al. [87] coined the term Shape Selectivity Index (SSI), which is defined as the yield ratio of p- and o-xylene observed on a shape-selective zeolite and extrapolated to zero conversion minus the same ratio obtained under identical experimental conditions on a catalyst with sufficiently large pores, i.e., in the complete absence of shape selectivity in m-xylene isomerization. Neither Dewing’s R value nor the Shape Selectivity Index by Joensen et al. received much attention by others.
A critical evaluation of the published data revealed [2] that a comparison of the rates of isomerization and disproportionation, if applied quantitatively to a larger number of zeolites, does not allow for a reliable ranking of largepore molecular sieves. No correlation at all exists between criterion (ii) and the window or pore size [2]. Therefore, criterion (ii) will not further be dealt with.
According to Martens et al. [88] there seems to be, however, very valuable information on the pore architecture in the distribution of the trimethylbenzenes formed from m-xylene in large-pore zeolites. If disproportionation
134 |
Y. Traa et al. |
occurs at all in 10-membered-ring zeolites, it gives 1,2,4-trimethylbenzene exclusively, probably because the diphenylmethane-type carbocation intermediate leading to this particular isomer can be best accommodated in their narrow pores. Exceptions are zeolites ZSM-50 (EUO) and NU-87 (NES) [89] in which 1,2,3- and 1,3,5-trimethylbenzene are formed as well. This can be rationalized if one assumes that the disproportionation takes place, at least in part, in the spacious side pockets running perpendicular to the 10-membered-ring channels (EUO) or in the 12-membered-ring channels (NES). For the zeolites with the largest pores or cavities, the distribution of the trimethylbenzenes is close to equilibrium. Hence, mechanistic, i.e., kinetic conclusions from these data should be drawn with great care, and therefore, criterion (iii) is not further considered here.
With respect to criterion (i), it has been concluded [2] that it allows for
a safe discrimination between 10-(Sp-xylene /So-xylene ≥ 2, S = selectivity) and 12-membered-ring (Sp-xylene /So-xylene ≈ 1.0 to 1.5) zeolites. However, later work revealed that the situation is more complex if the experimental condi-
tions for the test are not exactly defined and if one has non-uniform pore systems, as in the case of zeolite MCM-22 (MWW) (cf. Table 5 and cited references). This zeolite has two-dimensional sinusoidal channels (0.41 × 0.51 nm), large supercavities (0.71 nm diameter, 1.82 nm height) accessible through 10-membered ring openings (0.40 nm × 0.55 nm) and large hemicavities on the external surface (0.71 nm diameter, 0.70 nm depth).
As demonstrated by Laforge et al. [90], each of these pore systems affect
the Sp-xylene /So-xylene ratio entirely differently. These authors successively deactivated or poisoned the acid sites within each pore system to examine the
role they played during m-xylene transformation. Using this method, it was
shown that the external hemicavities gave an Sp-xylene /So-xylene ratio of 1.1 and a low selectivity to disproportionation. The supercavities, on the other
hand, gave an Sp-xylene /So-xylene ratio of 3.5. Furthermore, 98.5% of the disproportionation reactions and 35% of the isomerization reactions occurred
in the supercavities. In the sinusoidal pores, disproportionation was sterically
hindered and only isomerization resulted, giving an Sp-xylene /So-xylene ratio of 42.5. Of the m-xylene transformation that took place over MCM-22, 42% oc-
curred in the supercavities, 36% in the sinusoidal channels and 22% in the external hemicavities. Thus, the final product distribution is a combination of the contribution of all three pore systems, and one cannot speak of a single pore size. In addition, the acid sites in the different pore locations deactivated at different rates, altering the product distribution over time.
Thus, not surprisingly, the selectivity ratios Sp-xylene /So-xylene for zeolite MCM-22 reported in the literature differ greatly (cf. Table 5). Whereas Corma
et al. [92–94] and Adair et al. [95] determined their experimental data dependent on time on stream and extrapolated to zero time in order to account for the deactivation of the catalysts due to coking and their different decay rates, Ravishankar et al. [91] obtained the selectivity ratio after 2 h time on
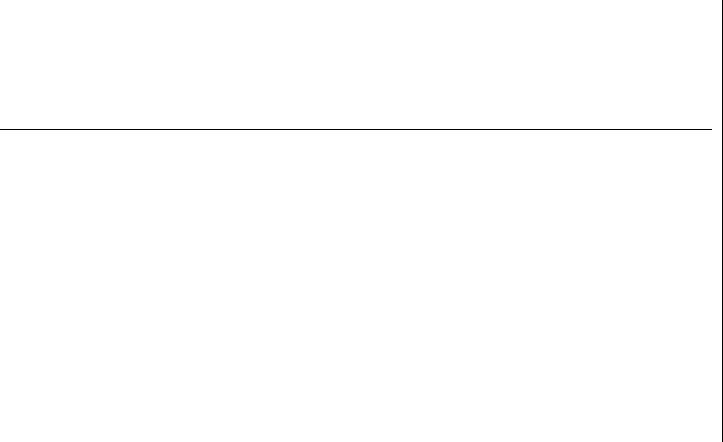
Table 5 Results from m-xylene reactions over zeolite H-MCM-22 (MWW) [90–95] (TOS = time on stream, X = conversion, S = selectivity)
Crystallite size |
nSi/nAl |
TOS/h |
T/K |
|
n˙H2 |
Xm-xylene |
|
Sp-xylene |
Refs. |
|
n˙m-xylene |
|
So-xylene |
||||||
|
|
|
|
|
|
|
|
||
1–2 µm |
14 |
2 |
573 |
4.0 |
58.1% |
1.03 |
[91] |
||
1–2 µm |
14 |
2 |
573 |
4.0 |
39.7% |
1.02 |
[91] |
||
1–2 µm |
14 |
2 |
573 |
4.0 |
20.2% |
1.06 |
[91] |
||
1–2 µm |
14 |
2 |
573 |
4.0 |
9.5% |
1.07 |
[91] |
||
1–2 µm |
14 |
2 |
573 |
4.0 |
4.1% |
0.87 |
[91] |
||
0.5 µm |
11 |
0 |
623 |
0 |
not given |
2.0 |
[92–94] |
||
submicrometer |
14 |
0 |
590 |
0 |
not given |
2.6 |
[95] |
||
not given |
10 |
0.03 |
623 |
0 |
13.3% |
3.8 |
[90] |
||
not given |
10 |
24 |
623 |
0 |
7.7% |
4.0 |
[90] |
||
|
|
|
|
|
|
|
|
|
|
Sieves Molecular of Size Pore the of Characterization
135

136 Y. Traa et al.
stream. However, since in the latter experiments hydrogen was present in the feed (as opposed to the former), deactivation might still be absent after 2 h time on stream. Possibly, the presence of hydrogen can account for the low
Sp-xylene /So-xylene ratio observed in [91].
Regarding the group of zeolites that provide access to the intrazeolitic pore system only via 10-membered-ring channels, but have significant space inside the pores due to large cavities (MWW), large side pockets (EUO) or 12-membered-ring bridges (NES), MCM-22 (MWW) and EU-1 (EUO) have selectivity ratios in the range of medium-pore zeolites (2.6 and 3.9, respectively), but the ratio of NU-87 (NES) is considerably lower (1.3 to 1.6, typical of large-pore zeolites) [89, 95] – which was explained by the high residence time of the isomers in the 12-membered-ring channels [89]. Corma et al. [96] also reported values typical of 12-membered-ring zeolites for zeolite NU-87. However, zeolite CIT-1 (CON), having 10and 12-membered-ring pore openings, was classified as medium-pore zeolite by Corma et al. [96], but as large-pore zeolite by Adair et al. [95]. More experimental data on the characteristics of more recently discovered zeolites in the m-xylene test reaction can be found in [25, 95–98].
The data discussed so far may lead one to conclude that the m-xylene test reaction is of limited use for probing the pore width of zeolites. However, Jones et al. [97] reported that the reactions of m-xylene could give, under proper experimental conditions, information enabling the characterization of medium-, large- and extra-large-pore zeolites with one and the same test reaction, whereas all other test reactions proposed so far are only appropriate for medium- or large-pore zeolites. These authors found that largeand extra- large-pore zeolites with one-dimensional channel systems, such as CIT-5
(CFI), SSZ-24 (AFI), SSZ-31 and UTD-1 (DON), have an Sp-xylene /So-xylene ratio < 1, whereas multidimensional zeolites such as zeolites Y (FAU) and
Beta (BEA) display ratios > 1 (note, however, that zeolite L (LTL) with unidimensional pores has a selectivity ratio > 1 as well). In addition, the authors stress that it is imperative that all zeolites be compared at exactly the same experimental conditions, for example, similar conversion levels and even the same flow rate (sufficiently high to ensure the absence of significant external diffusion limitations on reaction selectivity) are important. In addition, all zeolites should have roughly the same crystal size.
In conclusion, the m-xylene test reaction has found widespread application for probing the pore width of zeolites, probably because it is easy to handle (the number of products which have to be considered is small). However, this reaction cannot be applied blindly, especially if the zeolite possesses a non-uniform pore system. Used intelligently, perhaps together with selective poisoning, this reaction can provide valuable information on the role of various pore systems within a zeolite, played during a catalytic reaction. Furthermore, this could open a new perspective for the application of catalytic test reactions in modern catalysis research.
Characterization of the Pore Size of Molecular Sieves |
137 |
If properly handled, the test reaction might also allow for a meaningful classification of several classes of zeolite materials. However, a safe ranking of zeolites with respect to their pore width within these classes is certainly difficult and less safe.
4.2.3
Other Test Reactions
Csicsery [99, 100] recognized pronounced shape selectivity effects during the reaction of ortho-ethyltoluene in acidic zeolites. A wealth of information on the pore size of the catalyst can be deduced from the product distributions. Of course, all three criteria advanced for m-xylene conversion (cf. previous section) are applicable to Csicsery’s test as well. The latter furnishes, however, additional information, inter alia because two types of alkyl groups of different bulkiness can be transferred. Because of the larger size of orthoethyltoluene and the intermediates, transition states and products derived from it, this test might be much more appropriate for probing the pores of 12-membered-ring zeolites than the m-xylene test.
The disproportionation of ethylbenzene into benzene and the isomeric diethylbenzenes was originally proposed by Karge et al. [101] as a test reaction to collect rapid information on the number of strong Brønsted acid sites in zeolites. Later, the reaction was found suitable for probing the pore width as well. From comparative catalytic experiments with a variety of 10and 12- membered-ring zeolites [102, 103], the following criteria were established: (i) 12-membered-ring zeolites exhibit an induction period whilst 10-membered- ring zeolites do not; (ii) after the induction period, there is very little or no deactivation in 12-membered-ring zeolites, whereas in all 10-membered-ring zeolites, there is considerable deactivation from the very beginning of the catalytic experiment; (iii) on 12-membered-ring zeolites, the distribution of the diethylbenzenes is ca. 5 mol- % ortho-, 62 mol- % metaand 33 mol- % para-isomer; on 10-membered-ring zeolites, the ortho-isomer is often completely absent or it appears as a very minor product, especially at the onset of the catalytic experiment. The combined application of the above criteria allows for a safe discrimination between mediumand large-pore zeolites (for the structures examined so far), but not yet for a ranking of the zeolites according to their effective pore width. However, Das et al. [104] demonstrated that the p-diethylbenzene selectivity increased from 33.4% on H-ZSM-5 to 99.4% on silylated H-ZSM-5 and attributed this effect to the pore size regulation achieved by vapor deposition of the bulky tetraethyl orthosilicate followed by its calcination at 813 K. In a later study [105], the authors employed reaction mixtures containing 80% m-xylene and 20% ethylbenzene. With this mixture, essentially two reactions occurred on ZSM-5 zeolites at 678 K, namely m-xylene isomerization and ethylbenzene dealkylation to benzene and ethylene. With increasing silylation time, the m-xylene conversion

138 |
Y. Traa et al. |
decreased much more strongly than the ethylbenzene conversion. After a silylation period of 210 min, the conversion of m-xylene was zero, whereas the ethylbenzene conversion was still about 20%. This example shows that such test reactions can be useful for probing subtle differences in the pore size. However, they need to be designed, defined and refined for a small pore size range.
Kim et al. [106] proposed a test reaction particularly suitable for probing the effective pore width of large-pore zeolites. They chose a very bulky reactant, namely m-diisopropylbenzene, which has no access to 10-membered- ring pores, for the conversion with propene. Under the reaction conditions chosen, isomerization of m-diisopropylbenzene to its para-isomer and alkylation to the three isomeric triisopropylbenzenes occurred. It is the rationale in the design of this catalytic test reaction that 1,3,5-triisopropylbenzene is a bulkier product than its 1,2,4-isomer. A problem associated with this test reaction is the deactivation of the catalyst due to its direct exposure to an alkene under a substantial partial pressure. Nonetheless, with a sophisticated sampling strategy, from the selectivity ratio of the 1,3,5- to the 1,2,4-isomer, conclusions could be drawn on the effective pore width of the investigated materials that are in general agreement with their Spaciousness Indices (cf. Sect. 4.3.2). To minimize coke deposition in the catalyst pores, Singh et al. [107] proposed a modification of the test, i.e., the use of isopropanol instead of propene as alkylating agent and of a large excess of m-diisopropylbenzene and a solvent. Unfortunately, the authors restricted their studies to zeolites Y, mordenite and Beta, so that the complete and critical evaluation of this test reaction for probing extra-large-pore zeolites is still lacking.
The alkylation of biphenyl with propene has been suggested as a test reaction for examining the pore size of pillared clays, large-pore zeolites and related microporous materials [108]. The major selectivity criterion discussed is the content of o-isopropylbiphenyl in the monoalkylated product fraction. The reaction could have a much broader potential for characterizing largeand even extra-large-pore molecular sieves, but more systematic work on the influence of the pore size and geometry of the catalyst on the distribution of the mono-, diand, desirably, trialkylated products is needed.
An index analogous to the Constraint Index, yet designed for large-pore zeolites, was proposed by Miller [109]. He introduced the C8 Selectivity Index (C8 S.I.), defined as
ln(1 – Xn-Oc) |
|
|
C8S.I. ≡ ln(1 – X2,2,4-TM-Pn) |
, |
(3) |
as determined during competitive cracking of a 1 : 1 mixture of n-octane (n- Oc) and 2,2,4-trimethylpentane (2,2,4-TM-Pn) [109]. The rationale of this test is that n-octane can easily enter the pores, whereas 2,2,4-trimethylpentane cannot. This test is also suitable for bifunctional zeolites, since the effect of

Characterization of the Pore Size of Molecular Sieves |
139 |
the metal on the numerical value of the index has been demonstrated to be small [109].
Doblin et al. [110] proposed a more general reaction constraint index, namely the Selectivity Ratio (SR), defined as
log(1 – Xless branched isomer) |
|
|
SR ≡ log(1 – Xmore highly branched isomer) |
, |
(4) |
as determined during competitive cracking of a 1 : 1 mixture of two alkanes with the same number of carbon atoms. With this definition, Mobil’s Constraint Index (Sect. 4.2.1) becomes a special case of the Selectivity Ratio. (Note that, in the original papers of Miller [109] and Doblin et al. [110], the indices were formally defined in a slightly different manner which, from a chemical reaction engineering viewpoint, needed some re-definition.) Compared to the Constraint Index, the Selectivity Ratio is a more flexible index, since probe molecules can be chosen from a variety of alkanes to more closely match the catalyst pore size to be characterized. This is an interesting approach, but more experimental data collected on a much broader variety of zeolites including extra-large-pore materials is needed, before the usefulness of SR can be assessed.
Flego and Perego [111] recently proposed the aldol condensation of acetone as a more unusual test for characterizing both the acid site density and the pore dimension of small-, mediumand large-pore zeolites in their acidic forms. The products can be readily analyzed by UV/Vis spectroscopy. Medium-pore zeolites were shown to give phorone as the final product, whereas large-pore zeolites tend to favor the formation of isophorone. However, no quantitative criterion was coined, hence this test reaction appears to need more refinement, before it can be recommended for a more general application.
The last test reaction for acidic zeolites that has to be discussed is the “methanol conversion test”: The acid-catalyzed Methanol-to-Hydrocarbons (MTH) reaction is known to proceed in a stepwise manner [112, 113]: With increasing severity, dimethyl ether, olefins and a mixture of aromatics plus alkanes are successively formed. By suitable adjustment of the reaction conditions, either the yield of light olefins or that of aromatics plus alkanes can be maximized, the corresponding process variants being referred to as Methanol-to-Olefins (MTO) and Methanol-to-Gasoline (MTG), respectively. Large amounts of water are necessarily formed in the MTH reaction, and the risk exists that this brings about an undesired steaming of the zeolites with a concomitant framework dealumination. To minimize these effects, the zeolites to be tested are to be used in a form with a sufficiently high nSi/nAl ratio, yet a minimum amount of aluminum must be present in the framework since it generates the Brønsted acidity and, hence, the catalytic activity.
The “methanol conversion test” relies on the finding that the selectivity of the MTH reaction is strongly influenced by the zeolite pore geometry. Zeo-
140 |
Y. Traa et al. |
lite structures with 8-membered-ring pore apertures, such as erionite (ERI) or chabazite (CHA) are capable of converting methanol selectively to light olefins. Aromatics, if formed at the catalytic sites, would be trapped inside the large cavities existing in most of these small-pore zeolites. On mediumpore zeolites, such as ZSM-5, aromatics do occur in the product, but due to the space limitations inside the pores, the aromatics distribution terminates at around C10 (durene is widely considered as the bulkiest aromatic hydrocarbon occurring in an MTG product) with a maximum at around C8. In zeolite mordenite (MOR), the bulky polymethylbenzenes tend to be the main products, whereas in ZSM-12 (MTW) with its smaller effective pore diameter, a broader aromatics distribution has been observed [112]. These and other selectivity effects in the MTH reaction were exploited by Yuen et al. [114] for the characterization of AFI and CHA molecular sieves. Webster et al. [9] used the results from the test performed at different temperatures with zeolite H-ZSM-5 to assess the change of the effective pore dimension with temperature. The authors concluded that the effective channel size of H-ZSM-5 is between 0.662 and 0.727 nm at 300 ◦C, the MIN-2 (cf. Sect. 2.1) dimensions of the reaction products p-xylene (which is formed) and o-xylene (which is not formed), and at least 0.764 nm at 370 ◦C, the MIN-2 dimension of the reaction product 1,2,3-trimethylbenzene. Upon increasing the temperature, the dimensions of the channel intersections increase as well, namely from 0.817 by 0.888 nm to 0.908 by 0.909 nm [9].
Bendoraitis et al. [115] used a test reaction similar to methanol conversion (i.e., based on mass transfer shape selectivity) but this focused on reactant shape selectivity instead of product shape selectivity: These authors determined the catalytic pore sizes of ZSM-5, ZSM-23 (MTT) and mordenite on the basis of the sizes of molecules converted during the dewaxing of waxy distillate feeds to 0.55 by 0.70, 0.45 by 0.65 and 0.90 by 1.0 nm, respectively. As these examples show, this kind of test reaction can provide much useful information. However, the evaluation of the data is troublesome, since many products have to be considered and no criterion for an easy quantitative analysis has been defined. In addition, the crystal size of the materials examined should be kept constant, which is often difficult.
Mesoporous aluminosilicates assembled from microporous materials present a special challenge to the researcher because they have not one but two pore systems. How does one conduct catalytic test reactions on such catalysts in order to unequivocally determine the pore size? One way is to inactivate the acid sites in one of the pore systems, using an appropriate basic molecule, before studying the pores in the remaining system.
Sun et al. [116] used this method to study the microporosity in a series of ordered mesoporous aluminosilicates. First, the samples were treated using a bulky, basic organic molecule, namely 5,7-dibromo-8-hydroxy-quinoline. This molecule deactivated the acid sites in the mesopores but left those in the micropores untouched. Once the mesopores have been deactivated, the pore
Characterization of the Pore Size of Molecular Sieves |
141 |
size of the micropores can be established using shape-selective, catalytic test reactions in much the same way as described in the foregoing sections. Using carefully selected test reactions, Sun et al. [116] were able to demonstrate that the micropores of the mesoporous aluminosilicates are distinguishable from one another. Specifically, it was shown that the base-treated material Al-SBA-15 was catalytically active for 2-propanol dehydration but not for the cracking of cumene and 1,3,5-triisopropylbenzene (TIPB); the treated MAS-9 (assembled from ZSM-5 nanoclusters) was catalytically active for 2-propanol dehydration and cumene cracking but not for TIPB cracking, and the treated MAS-7 (Beta nanoclusters) was catalytically active for 2-propanol dehydration, cracking of cumene and cracking of TIPB. Thus using this method, it could be established that Al-SBA-15 contains micropores of 0.34 to 0.50 nm, MAS-9 micropores of 0.50 to 0.74 nm and MAS-7 micropores of 0.74 to 0.87 nm.
4.3
Test Reactions for Bifunctional Molecular Sieves
In typical bifunctional molecular sieves, the Brønsted acid form of a zeolite is modified by a small amount, i.e., typically 0.1 to 1 wt.- %, of a hydrogenation/dehydrogenation component, usually platinum or palladium. Since the early 1980s, the potential of catalytic reactions occurring on bifunctional forms of zeolites for characterizing the pore size and pore architecture of zeolites has been explored. Particular emphasis was placed on the isomerization and hydrocracking of long-chain n-alkanes, such as n-decane (cf. Sect. 4.3.1), and to hydrocracking of cycloalkanes, for example, butylcyclohexane (cf. Sect. 4.3.2). A factor these reactions have in common is that they are conducted under hydrogen, which is activated by the metal component of the catalyst. One very favorable repercussion of the presence of hydrogen is that coke formation and the concomitant catalyst deactivation are absent or very slow. This not only makes the experiments much easier (neither the conversion nor the selectivities vary with time on stream), but also eliminates the risk of progressively narrowing the pores by the deposition of carbonaceous residues formed in the test reaction.
The mechanisms of hydrocarbon conversion over bifunctional zeolite catalysts have been extensively discussed (cf., e.g., [2, 117–120]) and are beyond the scope of this chapter. In brief, the saturated hydrocarbon reactant is first dehydrogenated on the noble metal to the corresponding olefins which, in turn, are protonated at Brønsted acid sites. The resulting carbenium ions, while adsorbed at acid sites, undergo skeletal rearrangements and β-scissions. Finally, the product carbocations are desorbed from the acid sites as alkenes, which are hydrogenated on the noble metal. At low conversion, for example, monobranched cations, formed from n-alkanes in the first rearrangement step, will usually be readily desorbed and hydrogenated to isoalkanes, and these appear as the sole products. Upon raising the conver-
142 |
Y. Traa et al. |
sion, e.g., by increasing the residence time or the reaction temperature, the monobranched alkylcarbenium ions undergo another rearrangement. The resulting dibranched carbenium ions desorb and appear in the product as dibranched isoalkanes. Upon further increasing the conversion, the dibranched cations rearrange once more into tribranched ones. These can undergo the very rapid type A β-scission [120]. Its salient feature is that β-scission starts from a tertiary and leads again to a tertiary carbenium ion. Tertiary carbenium ions are much more stable than secondary or primary carbenium ions. Indeed, in the absence of shape selectivity, i.e., using a zeolite or any other catalyst with sufficiently spacious pores, the mechanism of hydrocracking of long-chain n-alkanes proceeds via this route: Several skeletal rearrangements are followed by type A β-scission; besides, there is a smaller contribution of type B β-scission [120] of dibranched carbenium ions, involving tertiary and the less stable secondary carbenium ions.
4.3.1
Isomerization and Hydrocracking of Long-Chain n-Alkanes
and Light (C7) Cycloalkanes – The Refined or Modified Constraint Index, CI
Whereas hydrocracking of n-alkanes proceeds via highly branched and, hence, relatively bulky alkylcarbenium ions in largeand extra-large-pore zeolites, the tribranched precursor ions of the favorable type A β-scission cannot form under the steric constraints imposed in medium-pore zeolites. The system is then forced into alternative routes via less bulky intermediates, i.e., the narrower the pores, the higher will be the contributions of β-scissions involving less stable secondary or even primary carbenium ions. This shift in the hydrocracking mechanism with decreasing pore width brings about a large number of selectivity changes in the hydrocracked product.
Striking shape selectivity effects do not only occur in hydrocracking, but also in skeletal isomerization, which precedes hydrocracking. If n-decane is converted on a bifunctional catalyst with sufficiently spacious pores, such as zeolite Pt/Ca-Y, the product mixture obtained at low conversion consists of all possible isodecanes with one branch, i.e., 2-, 3-, 4- and 5-methylnonane, 3- and 4-ethyloctane as well as 4-propylheptane [121]. As the catalyst pores become narrower, the bulkier isomers cannot be formed any more inside the pores or cannot escape from there. On Pt/H-ZSM-5, for example, neither 4-propylheptane nor the two ethyloctane isomers are formed [120, 122].
An even more subtle shape selectivity effect concerns the formation of the four isomeric methylnonanes. In the absence of shape selectivity and at low conversions, i.e., in the kinetic regime, 2- and 5-methylnonane form about half as fast as 3- and 4-methylnonane. This can be readily understood in terms of the branching mechanism via protonated cyclopropanes, cf. [121]. 2-Methylnonane is the kinetically preferred isomer in 10-membered-ring zeolites. Based on the observation that, with decreasing pore width of the