
Matta, Boyd. The quantum theory of atoms in molecules
.pdfPart IV
Chemical Bonding and Reactivity

345
13
Interactions Involving Metals:
From ‘‘Chemical Categories’’ to QTAIM, and Backwards
Piero Macchi and Angelo Sironi
13.1 Introduction
The Lewis model [1] enables description of bonds between main group elements but its extension to the realm of transition metal compounds is di cult because many subtle e ects complicate solution of the chemical bonding puzzle. Interpretation is equally uncertain for weak intermolecular interactions. Similarly, the quantum theory of atoms in molecules (QTAIM) [2] readily visualizes the intuitive concepts of atoms and bonds whereever the Lewis theory works whereas for transition metal complexes the topology of the electron density r(r) reveals chemical bonding features in a less straightforward manner.
More e ort is therefore necessary to build up the ‘‘correspondence rules’’ linking QTAIM to traditional bonding concepts. As soon as a proper model is devised, however, significant information can be retrieved, resulting in new understanding or even some re-thought of ‘‘classical’’ chemical bonds involving transition metals. This is the objective of this chapter. Some examples from the recent literature will be discussed. To enable unbiased comparison we reproduce all theoretical calculations at the same level of theory, B3LYP/6-311þþG(2d,2p), except for metal hydride bridges, for which it has been demonstrated [3] that B3LYP [4] functional is less reliable than BP86 [5].
13.2
The Electron Density in Isolated Metal Atoms – Hints of Anomalies
What is the electron density of an isolated atom telling us about that atom? Many atomic properties can be predicted from a knowledge of the distribution of the ground-state electron density of the isolated element. For example, the concentration of the valence electrons, and their separation from the core, can be used to predict bond distances, the tendency to form covalent bonding, the degree of localization of the bonding electrons, etc.

346 13 Interactions Involving Metals: From ‘‘Chemical Categories’’ to QTAIM, and Backwards
Fig. 13.1 H(r) (solid line) and L(r) (bold line) radial profiles of the isolated N (a), Mn (b) and As (c) atoms. r is in A˚ , H(r) and L(r) are in a.u. (left scale for L, right scale for H). L(r) of As has a ripple maximum of its outermost shell at ca. r ¼ 1:3 A˚ (this is missing in Mn).
As pointed out by Bader [2, 6], the negative Laplacian (L(r) ¼ ‘2r(r)) of an isolated ground-state atomic density [7] reproduces the electronic shell structure, alternating positive and negative regions with a maximum and a minimum for each shell [8]. However, heavy atoms deviate from this behavior [9]: the expected maxima and minima of the N shell are not observed for the elements from Sc to Ge, but from As to Kr the M and N shells are again separated, although the outermost maxima do not necessarily have L(r) > 0 (the radial L(r) for N, Mn, and As are shown in Fig. 13.1). Similar trends are observed for the elements of successive rows and have been justified on the basis of the di use character of the outermost electrons [10b]. The electron density r(r) decreases regularly to zero, as also do the kinetic energy density G(r) and the potential energy density V(r) in absolute values (being everywhere negative). The total energy density H(r) (¼ V(r) þ G(r)) is not monotonic, however, although it does not have the same structure as L(r).
Because of to the local virial theorem [2]:
ðh2=4mÞ‘2rðrÞ ¼ 2GðrÞ þ VðrÞ |
ð1Þ |
H(r) can be rewritten as:
HðrÞ ¼ GðrÞ þ VðrÞ ¼ 1=2ððh2=4mÞ‘2rðrÞ þ VðrÞÞ |
ð2Þ |
showing it is somewhat reminiscent of the features of the Laplacian distribution: an atom usually has an inner (core) region and an outer (valence) shell, irre-
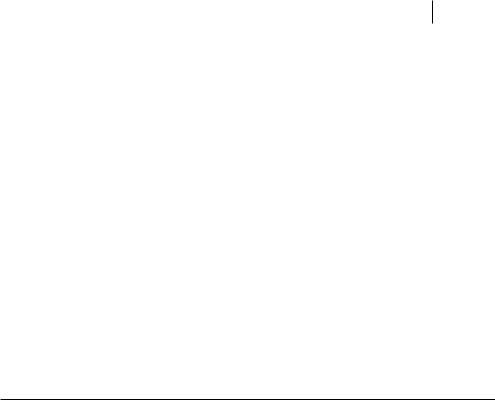
13.2 The Electron Density in Isolated Metal Atoms – Hints of Anomalies 347
spective of its principal quantum number. The boarders might occur at H(r) > 0 and the ‘‘atomic’’ limit at large r (Fig. 13.1).
When a chemical bond is formed, all these functions are no longer spherical and their properties characterize atomic interactions. In particular, L(r) enhances the features of the charge distribution and electron pair localization, providing a physical connection with the classical Lewis model and the valence shell electronpair repulsion theory [11]. L(r), however, remains a ected by the distribution in the unperturbed atom. For example, when a covalent bond links two second-row atoms, each has a distinct valence shell charge concentration (VSCC, maxima of L(r)) along the bond path, quite close to the location of the corresponding L(r) valence shell maxima in the isolated atomic density. Because the outermost shells of the two bonded atoms partially overlap, we have L(r) > 0 in the bond region, typical of an open-shell interaction. Naively, this enables visualization of the electron sharing mechanism; this is, however, better explained physically by means of the bond delocalization index (d) [12, 13] related to the electron pairs shared between two atoms (Table 13.1). Along the bond path, V(r) dominates G(r), hence H(r) < 0. The regions where the electrons are localized are also visible in the H(r) distribution, see for example Fig. 13.2a, in which the H(r) distribution of C2H6 is
Properties of the electron-density distribution in some reference molecules discussed in the text.
Compound |
Bond, |
dAxB |
dA-bcp |
dB-bcp |
r |
‘2r |
Hb/r |
b |
Gb/r |
AXB |
r(r) |
d(A, B) |
|
|
|
|
|
b |
b |
|
b |
|
|
||
|
A–B |
(A˚ )[a] |
(A˚ )[a] |
(A˚ )[a] |
(e A˚C3) |
(e A˚C5) |
(h eC1) |
(h eC1) |
Þ(e A˚C1)[b] |
[SCF][c] |
||
H2 |
HaH |
0.743 |
0.371 |
0.371 |
1.822 |
29.2 |
1.12 |
0.00 |
1.42 |
|
1.00 |
|
C2H6 |
CaC |
1.529 |
0.765 |
0.765 |
1.597 |
11.9 |
0.75 |
0.23 |
2.15 |
|
1.00 |
|
C2H4 |
CbC |
1.326 |
0.663 |
0.663 |
2.381 |
27.1 |
1.19 |
0.39 |
2.98 |
|
1.89 |
|
NaF |
NaaF |
1.942 |
0.902 |
1.040 |
0.342 |
9.92 |
0.27 |
1.76 |
0.53 |
|
0.27 |
|
Ne2 |
NeaNe |
3.795 |
1.898 |
1.898 |
0.002 |
0.06 |
0.86 |
1.18 |
0.004 |
0.002 |
||
CO |
CcO |
1.126 |
0.386 |
0.740 |
3.416 |
5.31 |
1.92 |
2.03 |
3.23 |
|
1.81 |
|
H3BCO |
BaC |
1.521 |
0.495 |
1.025 |
1.004 |
10.42 |
0.85 |
1.58 |
2.06 |
|
0.47 |
|
H3BCO |
CcO |
1.130 |
0.390 |
0.740 |
3.366 |
4.87 |
1.89 |
1.99 |
3.21 |
|
1.64 |
|
Cr(CO)6 |
CraC |
1.927 |
0.952 |
0.976 |
0.717 |
11.2 |
0.28 |
1.37 |
2.12 |
|
0.83 |
|
Cr(CO)6 |
CcO |
1.140 |
0.392 |
0.748 |
3.291 |
3.09 |
1.89 |
1.96 |
3.23 |
|
1.62 |
|
Co2(CO)6(NH3)2 |
CoaN |
2.063 |
0.973 |
1.090 |
0.502 |
8.40 |
0.14 |
1.31 |
1.42 |
|
0.51 |
|
Co2(CO)6(PH3)2 |
CoaP |
2.190 |
1.013 |
1.177 |
0.628 |
4.10 |
0.34 |
0.80 |
1.98 |
|
0.81 |
|
Co2(CO)6(AsH3)2 |
CoaAs |
2.320 |
1.044 |
1.277 |
0.512 |
3.52 |
0.30 |
0.78 |
1.76 |
|
0.71 |
|
Na2 |
NaaNa |
3.052 |
1.526 |
1.526 |
0.063 |
0.03 |
0.13 |
0.10 |
0.52 |
|
1.00 |
|
Co2(CO)6(AsH3)2 |
CoaCo |
2.693 |
1.346 |
1.346 |
0.244 |
0.10 |
0.30 |
0.33 |
1.58 |
|
0.52 |
a For each bond, dAaB, dA-bcp, and dB-bcp are the A–B interatomic distances
and the distances of atoms A and B, respectively, from the bcp.
Þ
b AXB rðrÞ is the electron density integrated over the (A|B) interatomic surface. c d(A, B) is the delocalization index between atoms A and B.

348 13 Interactions Involving Metals: From ‘‘Chemical Categories’’ to QTAIM, and Backwards
Fig. 13.2 H(r) distributions in (a) C2H6, (b) NaF, (c) Ne2, (d) CO (contours increase in value in the orderGx 10n a.u., where
x ¼ 1:0; 2:0; 4:0; 8:0 and 3 an aþ2; solid lines are negative contours). Bold labels and dots represent atoms lying in the plane; empty symbols represent atoms projected on to the plot plane. IAS and molecular graphs are superimposed.
shown. The topology of L(r) defines the so called atomic graph around each atom in a molecule, which typically reproduces its hybridization state [2].
If, in contrast, an ionic bond is created, as in NaF, the outermost shells of the cation and the anion are superimposed. The bcp is shifted towards Na in a large and flat negative region of L(r) where r(r) is small (Table 13.1). The electronic configurations of F and Na now coincide with that of Ne and are better approximated by two closed shells. Only a small electron delocalization is actually observed at the bcp and G(r) dominates in the valence region; thus a large area of H(r) > 0 separates the two atoms (Fig. 13.2b).
Although the correlation between L(r) and covalent interactions seems valid, Cremer and Kraka [14] were the first to note it could not su ce for weaker covalent bonds and, instead, they proposed investigating whether the electron density in the bonding region stabilized the system, for example by analyzing H(r) at the bcp or over the whole interatomic surface. The implicit assumption is that molec-

13.3 Two-center Bonding 349
ular density would be defined by the boundary of H(r), an idea more recently applied by Tsirelson [15], who reconstructed the energy-density plots in ionic and molecular crystals from experimentally derived electron density, by using DFT-like approximations to compute G(r) [15, 16], hence H(r), by use of Eq. (1). The plots in Fig. 13.2 demonstrate that the intuition of Cremer and Kraka was probably correct. This approach can be even more useful when applied to transition metal chemistry.
Bader also considered the energy-density quantities in detail, suggesting that H(r), G(r), and V(r) be measured on the basis of the total amount of electron density, with which they clearly correlate. Indicators such as Hb/rb or Gb/rb thus inform us whether the potential or kinetic energy density is dominating at the bcp or whether G(r) is in local excess with respect to the electron density itself. Gr/rr usually increases in correspondence with a ‘‘shell closure’’ – ‘‘external’’ if two closed-shell atoms are interacting or ‘‘internal’’ at short interatomic distances, for example in multiple bonds, in which the two cores become close (this is discussed in more detail elsewhere [17]).
When the bond is covalent but is significantly polarized the interatomic surface is shifted toward the VSCC of the least electronegative atom and, eventually, both VSCCs belong to the basin of the most electronegative atom. In CO the bcp is so close to C that ‘2rb > 0 and Gb/rb > 0. Nevertheless, the H(r) is everywhere negative (Fig. 13.2). The shift of the bcp significantly a ects the delocalization index between C and O, which no longer represents the expected number of Lewis bonded pairs (Table 13.1), counting of which in the presence of charge transfer is not possible solely on the basis of the pair density [13].
13.3
Two-center Bonding
As introduced in the previous section, the chemistry of main-group elements is usually characterized by ionic or covalent bonds. The latter are typically discussed in terms of interactions between hybridized atomic orbitals, in which each binding partner contributes one electron to the bonding pair. This picture is substantially valid for metal–metal bonds also, but according to the most popular theories on transition metal chemistry (for example the ligand-field theory, LFT) [18], di erent genealogy is required when describing two-center (2c) metal– ligand bonding [19] resulting from interaction of doubly occupied orbitals on the ligand and empty orbitals on the metal (donation) or vice versa (back-donation).
A di erent viewpoint was proposed by Bersuker [20], who assigned the nature of two-center–two-electron (2c–2e) bonds without resorting to the actual parenthood of the electrons involved in the bonding but di erentiating between valence (localized) and coordination (delocalized) bonds. This scheme is, apparently, closer to the actual phenomenon of the electron density because, in principle, when a chemical bond is formed the participating electrons lose their original affiliation. ‘‘However, the genealogic concepts seems to be quite convoluted also

350 13 Interactions Involving Metals: From ‘‘Chemical Categories’’ to QTAIM, and Backwards
within the framework of QTAIM, because the bonding to transition metals is characterised by a ‘‘low overlap’’ [18] and thus quite reminiscent of the electron density distribution at atomic level.’’
Following the genealogy scheme, we analyze two di erent 2c bonds to a metal: the classical coordination of a ligand (i.e. the donation of one electron pair) and metal–metal bonding (i.e. pairing of two electrons).
13.3.1
The Dative Bond
A classical donor–acceptor bond involving main-group atoms is, for example, OCaBH3 [21]. The Laplacian distribution, the bond paths, and the interatomic surfaces are shown in Fig. 13.3a. A unique VSCC, corresponding to the lone pair of the nucleophilic atom (C) and charge depletion at the electrophilic atom (B) are found along the bond path. The interatomic surface is shifted toward the acceptor and lies inside the charge-depletion shell of B. Therefore, ‘2rb > 0, although significant deformation has occurred relative to the constituting molecular frag-
Fig. 13.3 ‘2r(r) and H(r) distributions in H3BCO (a and b) and (CO)5Cr(CO) (c and d). Contours and labeling are as in Fig. 13.2.

13.3 Two-center Bonding 351
ments. The presence of some polarization undermines a simple electrostatic model for this bond. In fact, although G(r) is certainly large, it does not overwhelm V(r), hence H(r) has a negative continuum between B and C (Fig. 13.3b). As anticipated above, interactions in which polarity plays an important role are characterized by significantly reduced delocalization indexes, d(B, C) ¼ 0:47.
From a di erent perspective, some energy partitioning schemes [22] predicted that the electrostatic energy a ords the largest stabilization in these complexes. It is conceivable that the electrostatic and the covalent terms are closely connected and mutually reinforced, and thus more di cult to separate by analyzing r(r). H(r), however, cannot inform us which e ect dominates but rather on the occurrence of some stabilization as a result of orbital interaction [14].
No inverse e ect (i.e. from B to C) is observable, because the CaO bond indicators (including the distance) are not a ected by the coordination and delocalization between O and B is very small (d ¼ 0:03). There is, however, some electron sharing between the C and the H atoms (d ¼ 0:24), because of the delocalized nature of the bonding inside the BH3 unit.
13.3.1.1 Metal Carbonyls
The binary transition-metal carbonyl complexes M(CO)n are the most studied organometallic molecules, and are often used as reference materials to describe the bonding of transition metals in low oxidation states. The bonding in Cr(CO)6, Fe(CO)5, and Ni(CO)4 is usually understood in terms of the Dewar–Chatt– Duncanson mechanism [23], which invokes reciprocal donation of electron pairs (one from the C lone pair and one from correctly oriented metal d orbitals). The electrons used in the bonding are quite localized in nonbonded regions of the isolated unperturbed fragments, i.e. M(CO)n 1 and CO. Because of the abovementioned genealogy, this localization is only marginally a ected by the formation of a bond (Fig. 13.3c). Electron delocalization between the metal and the carbonyl is, in fact, approximately 1.0 (Table 13.1), even though more than one electron pair is formally involved in the bonding.
The MaC interatomic surface lies in a region of charge depletion even more pronounced than that in OCaBH3, although associated with H(r) < 0 (Fig. 13.3d). Again the dichotomy between covalent and electrostatic interaction is mixed, without easy deconvolution [24].
The three complexes introduced above are also representative of three di erent types of stereochemistry (octahedral, bipyramidal, and tetrahedral) and electronic configurations (d6, d8 and d10) of zerovalent metals. In LFT terms they correspond to di erent splitting of d-orbitals (t2g/eg; e00/e0/a1; eg/t2g) [18], which a ects L(r) distribution of the isolated metals in their corresponding electronic configuration [10]. The atomic graphs of the metals predicted at theoretical level and those derived from accurate experimental electron density determination were compared in a recent study [25]. In Cr(CO)6 the atomic graph of Cr is cubic in shape [eight vertexes, twelve edges, and six faces]. The eight vertexes are ð3; 3Þ critical points of L(r) (i.e. charge accumulations produced by the t2g orbitals) and the six faces are centered by ð3; þ3Þ critical points (i.e. charge depletion