
- •CONTENTS
- •Preface
- •Contributors
- •1 Introduction to Toxicology
- •1.1 Definition and Scope, Relationship to Other Sciences, and History
- •1.1.2 Relationship to Other Sciences
- •1.1.3 A Brief History of Toxicology
- •1.3 Sources of Toxic Compounds
- •1.3.1 Exposure Classes
- •1.3.2 Use Classes
- •1.4 Movement of Toxicants in the Environment
- •Suggested Reading
- •2.1 Introduction
- •2.2 Cell Culture Techniques
- •2.2.1 Suspension Cell Culture
- •2.2.2 Monolayer Cell Culture
- •2.2.3 Indicators of Toxicity in Cultured Cells
- •2.3 Molecular Techniques
- •2.3.1 Molecular Cloning
- •2.3.2 cDNA and Genomic Libraries
- •2.3.3 Northern and Southern Blot Analyses
- •2.3.4 Polymerase Chain Reaction (PCR)
- •2.3.5 Evaluation of Gene Expression, Regulation, and Function
- •2.4 Immunochemical Techniques
- •Suggested Reading
- •3.1 Introduction
- •3.2 General Policies Related to Analytical Laboratories
- •3.2.1 Standard Operating Procedures (SOPs)
- •3.2.2 QA/QC Manuals
- •3.2.3 Procedural Manuals
- •3.2.4 Analytical Methods Files
- •3.2.5 Laboratory Information Management System (LIMS)
- •3.3 Analytical Measurement System
- •3.3.1 Analytical Instrument Calibration
- •3.3.2 Quantitation Approaches and Techniques
- •3.4 Quality Assurance (QA) Procedures
- •3.5 Quality Control (QC) Procedures
- •3.6 Summary
- •Suggested Reading
- •4 Exposure Classes, Toxicants in Air, Water, Soil, Domestic and Occupational Settings
- •4.1 Air Pollutants
- •4.1.1 History
- •4.1.2 Types of Air Pollutants
- •4.1.3 Sources of Air Pollutants
- •4.1.4 Examples of Air Pollutants
- •4.1.5 Environmental Effects
- •4.2 Water and Soil Pollutants
- •4.2.1 Sources of Water and Soil Pollutants
- •4.2.2 Examples of Pollutants
- •4.3 Occupational Toxicants
- •4.3.1 Regulation of Exposure Levels
- •4.3.2 Routes of Exposure
- •4.3.3 Examples of Industrial Toxicants
- •Suggested Reading
- •5 Classes of Toxicants: Use Classes
- •5.1 Introduction
- •5.2 Metals
- •5.2.1 History
- •5.2.2 Common Toxic Mechanisms and Sites of Action
- •5.2.3 Lead
- •5.2.4 Mercury
- •5.2.5 Cadmium
- •5.2.6 Chromium
- •5.2.7 Arsenic
- •5.2.8 Treatment of Metal Poisoning
- •5.3 Agricultural Chemicals (Pesticides)
- •5.3.1 Introduction
- •5.3.3 Organochlorine Insecticides
- •5.3.4 Organophosphorus Insecticides
- •5.3.5 Carbamate Insecticides
- •5.3.6 Botanical Insecticides
- •5.3.7 Pyrethroid Insecticides
- •5.3.8 New Insecticide Classes
- •5.3.9 Herbicides
- •5.3.10 Fungicides
- •5.3.11 Rodenticides
- •5.3.12 Fumigants
- •5.3.13 Conclusions
- •5.4 Food Additives and Contaminants
- •5.5 Toxins
- •5.5.1 History
- •5.5.2 Microbial Toxins
- •5.5.3 Mycotoxins
- •5.5.4 Algal Toxins
- •5.5.5 Plant Toxins
- •5.5.6 Animal Toxins
- •5.6 Solvents
- •5.7 Therapeutic Drugs
- •5.8 Drugs of Abuse
- •5.9 Combustion Products
- •5.10 Cosmetics
- •Suggested Reading
- •6 Absorption and Distribution of Toxicants
- •6.1 Introduction
- •6.2 Cell Membranes
- •6.3 Mechanisms of Transport
- •6.3.1 Passive Diffusion
- •6.4 Physicochemical Properties Relevant to Diffusion
- •6.4.1 Ionization
- •6.5 Routes of Absorption
- •6.5.1 Extent of Absorption
- •6.5.2 Gastrointestinal Absorption
- •6.5.3 Dermal Absorption
- •6.5.4 Respiratory Penetration
- •6.6 Toxicant Distribution
- •6.6.1 Physicochemical Properties and Protein Binding
- •6.7 Toxicokinetics
- •Suggested Reading
- •7 Metabolism of Toxicants
- •7.1 Introduction
- •7.2 Phase I Reactions
- •7.2.4 Nonmicrosomal Oxidations
- •7.2.5 Cooxidation by Cyclooxygenases
- •7.2.6 Reduction Reactions
- •7.2.7 Hydrolysis
- •7.2.8 Epoxide Hydration
- •7.2.9 DDT Dehydrochlorinase
- •7.3 Phase II Reactions
- •7.3.1 Glucuronide Conjugation
- •7.3.2 Glucoside Conjugation
- •7.3.3 Sulfate Conjugation
- •7.3.4 Methyltransferases
- •7.3.7 Acylation
- •7.3.8 Phosphate Conjugation
- •Suggested Reading
- •8 Reactive Metabolites
- •8.1 Introduction
- •8.2 Activation Enzymes
- •8.3 Nature and Stability of Reactive Metabolites
- •8.4 Fate of Reactive Metabolites
- •8.4.1 Binding to Cellular Macromolecules
- •8.4.2 Lipid Peroxidation
- •8.4.3 Trapping and Removal: Role of Glutathione
- •8.5 Factors Affecting Toxicity of Reactive Metabolites
- •8.5.1 Levels of Activating Enzymes
- •8.5.2 Levels of Conjugating Enzymes
- •8.5.3 Levels of Cofactors or Conjugating Chemicals
- •8.6 Examples of Activating Reactions
- •8.6.1 Parathion
- •8.6.2 Vinyl Chloride
- •8.6.3 Methanol
- •8.6.5 Carbon Tetrachloride
- •8.6.8 Acetaminophen
- •8.6.9 Cycasin
- •8.7 Future Developments
- •Suggested Reading
- •9.1 Introduction
- •9.2 Nutritional Effects
- •9.2.1 Protein
- •9.2.2 Carbohydrates
- •9.2.3 Lipids
- •9.2.4 Micronutrients
- •9.2.5 Starvation and Dehydration
- •9.2.6 Nutritional Requirements in Xenobiotic Metabolism
- •9.3 Physiological Effects
- •9.3.1 Development
- •9.3.2 Gender Differences
- •9.3.3 Hormones
- •9.3.4 Pregnancy
- •9.3.5 Disease
- •9.3.6 Diurnal Rhythms
- •9.4 Comparative and Genetic Effects
- •9.4.1 Variations Among Taxonomic Groups
- •9.4.2 Selectivity
- •9.4.3 Genetic Differences
- •9.5 Chemical Effects
- •9.5.1 Inhibition
- •9.5.2 Induction
- •9.5.3 Biphasic Effects: Inhibition and Induction
- •9.6 Environmental Effects
- •9.7 General Summary and Conclusions
- •Suggested Reading
- •10 Elimination of Toxicants
- •10.1 Introduction
- •10.2 Transport
- •10.3 Renal Elimination
- •10.4 Hepatic Elimination
- •10.4.2 Active Transporters of the Bile Canaliculus
- •10.5 Respiratory Elimination
- •10.6 Conclusion
- •Suggested Reading
- •11 Acute Toxicity
- •11.1 Introduction
- •11.2 Acute Exposure and Effect
- •11.3 Dose-response Relationships
- •11.4 Nonconventional Dose-response Relationships
- •11.5 Mechanisms of Acute Toxicity
- •11.5.1 Narcosis
- •11.5.2 Acetylcholinesterase Inhibition
- •11.5.3 Ion Channel Modulators
- •11.5.4 Inhibitors of Cellular Respiration
- •Suggested Reading
- •12 Chemical Carcinogenesis
- •12.1 General Aspects of Cancer
- •12.2 Human Cancer
- •12.2.1 Causes, Incidence, and Mortality Rates of Human Cancer
- •12.2.2 Known Human Carcinogens
- •12.3 Classes of Agents Associated with Carcinogenesis
- •12.3.2 Epigenetic Agents
- •12.4 General Aspects of Chemical Carcinogenesis
- •12.5 Initiation-Promotion Model for Chemical Carcinogenesis
- •12.6 Metabolic Activation of Chemical Carcinogens and DNA Adduct Formation
- •12.7 Oncogenes
- •12.8 Tumor Suppressor Genes
- •12.8.1 Inactivation of Tumor Suppressor Genes
- •12.8.2 p53 Tumor Suppressor Gene
- •12.9 General Aspects of Mutagenicity
- •12.10 Usefulness and Limitations of Mutagenicity Assays for the Identification of Carcinogens
- •Suggested Reading
- •13 Teratogenesis
- •13.1 Introduction
- •13.2 Principles of Teratology
- •13.3 Mammalian Embryology Overview
- •13.4 Critical Periods
- •13.5 Historical Teratogens
- •13.5.1 Thalidomide
- •13.5.2 Accutane (Isotetrinoin)
- •13.5.3 Diethylstilbestrol (DES)
- •13.5.4 Alcohol
- •13.6 Testing Protocols
- •13.6.1 FDA Guidelines for Reproduction Studies for Safety Evaluation of Drugs for Human Use
- •13.6.3 Alternative Test Methods
- •13.7 Conclusions
- •Suggested Reading
- •14 Hepatotoxicity
- •14.1 Introduction
- •14.1.1 Liver Structure
- •14.1.2 Liver Function
- •14.2 Susceptibility of the Liver
- •14.3 Types of Liver Injury
- •14.3.1 Fatty Liver
- •14.3.2 Necrosis
- •14.3.3 Apoptosis
- •14.3.4 Cholestasis
- •14.3.5 Cirrhosis
- •14.3.6 Hepatitis
- •14.3.7 Oxidative Stress
- •14.3.8 Carcinogenesis
- •14.4 Mechanisms of Hepatotoxicity
- •14.5 Examples of Hepatotoxicants
- •14.5.1 Carbon Tetrachloride
- •14.5.2 Ethanol
- •14.5.3 Bromobenzene
- •14.5.4 Acetaminophen
- •14.6 Metabolic Activation of Hepatotoxicants
- •Suggested Reading
- •15 Nephrotoxicity
- •15.1 Introduction
- •15.1.1 Structure of the Renal System
- •15.1.2 Function of the Renal System
- •15.2 Susceptibility of the Renal System
- •15.3 Examples of Nephrotoxicants
- •15.3.1 Metals
- •15.3.2 Aminoglycosides
- •15.3.3 Amphotericin B
- •15.3.4 Chloroform
- •15.3.5 Hexachlorobutadiene
- •Suggested Reading
- •16 Toxicology of the Nervous System
- •16.1 Introduction
- •16.2 The Nervous system
- •16.2.1 The Neuron
- •16.2.2 Neurotransmitters and their Receptors
- •16.2.3 Glial Cells
- •16.3 Toxicant Effects on the Nervous System
- •16.3.1 Structural Effects of Toxicants on Neurons
- •16.3.2 Effects of Toxicants on Other Cells
- •16.4 Neurotoxicity Testing
- •16.4.1 In vivo Tests of Human Exposure
- •16.4.2 In vivo Tests of Animal Exposure
- •16.4.3 In vitro Neurochemical and Histopathological End Points
- •16.5 Summary
- •Suggested Reading
- •17 Endocrine System
- •17.1 Introduction
- •17.2 Endocrine System
- •17.2.1 Nuclear Receptors
- •17.3 Endocrine Disruption
- •17.3.1 Hormone Receptor Agonists
- •17.3.2 Hormone Receptor Antagonists
- •17.3.3 Organizational versus Activational Effects of Endocrine Toxicants
- •17.3.4 Inhibitors of Hormone Synthesis
- •17.3.5 Inducers of Hormone Clearance
- •17.3.6 Hormone Displacement from Binding Proteins
- •17.4 Incidents of Endocrine Toxicity
- •17.4.1 Organizational Toxicity
- •17.4.2 Activational Toxicity
- •17.4.3 Hypothyroidism
- •17.5 Conclusion
- •Suggested Reading
- •18 Respiratory Toxicity
- •18.1 Introduction
- •18.1.1 Anatomy
- •18.1.2 Cell Types
- •18.1.3 Function
- •18.2 Susceptibility of the Respiratory System
- •18.2.1 Nasal
- •18.2.2 Lung
- •18.3 Types of Toxic Response
- •18.3.1 Irritation
- •18.3.2 Cell Necrosis
- •18.3.3 Fibrosis
- •18.3.4 Emphysema
- •18.3.5 Allergic Responses
- •18.3.6 Cancer
- •18.3.7 Mediators of Toxic Responses
- •18.4 Examples of Lung Toxicants Requiring Activation
- •18.4.1 Introduction
- •18.4.2 Monocrotaline
- •18.4.3 Ipomeanol
- •18.4.4 Paraquat
- •18.5 Defense Mechanisms
- •Suggested Reading
- •19 Immunotoxicity
- •19.1 Introduction
- •19.2 The Immune System
- •19.3 Immune Suppression
- •19.4 Classification of Immune-Mediated Injury (Hypersensitivity)
- •19.5 Effects of Chemicals on Allergic Disease
- •19.5.1 Allergic Contact Dermatitis
- •19.5.2 Respiratory Allergens
- •19.5.3 Adjuvants
- •19.6 Emerging Issues: Food Allergies, Autoimmunity, and the Developing Immune System
- •Suggested Reading
- •20 Reproductive System
- •20.1 Introduction
- •20.2 Male Reproductive Physiology
- •20.3 Mechanisms and Targets of Male Reproductive Toxicants
- •20.3.1 General Mechanisms
- •20.3.2 Effects on Germ Cells
- •20.3.3 Effects on Spermatogenesis and Sperm Quality
- •20.3.4 Effects on Sexual Behavior
- •20.3.5 Effects on Endocrine Function
- •20.4 Female Reproductive Physiology
- •20.5 Mechanisms and Targets of Female Reproductive Toxicants
- •20.5.1 Tranquilizers, Narcotics, and Social Drugs
- •20.5.2 Endocrine Disruptors (EDs)
- •20.5.3 Effects on Germ Cells
- •20.5.4 Effects on the Ovaries and Uterus
- •20.5.5 Effects on Sexual Behavior
- •Suggested Reading
- •21 Toxicity Testing
- •21.1 Introduction
- •21.2 Experimental Administration of Toxicants
- •21.2.1 Introduction
- •21.2.2 Routes of Administration
- •21.3 Chemical and Physical Properties
- •21.4 Exposure and Environmental Fate
- •21.5 In vivo Tests
- •21.5.1 Acute and Subchronic Toxicity Tests
- •21.5.2 Chronic Tests
- •21.5.3 Reproductive Toxicity and Teratogenicity
- •21.5.4 Special Tests
- •21.6 In vitro and Other Short-Term Tests
- •21.6.1 Introduction
- •21.6.2 Prokaryote Mutagenicity
- •21.6.3 Eukaryote Mutagenicity
- •21.6.4 DNA Damage and Repair
- •21.6.5 Chromosome Aberrations
- •21.6.6 Mammalian Cell Transformation
- •21.6.7 General Considerations and Testing Sequences
- •21.7 Ecological Effects
- •21.7.1 Laboratory Tests
- •21.7.2 Simulated Field Tests
- •21.7.3 Field Tests
- •21.8 Risk Analysis
- •21.9 The Future of Toxicity Testing
- •Suggested Reading
- •22 Forensic and Clinical Toxicology
- •22.1 Introduction
- •22.2 Foundations of Forensic Toxicology
- •22.3 Courtroom Testimony
- •22.4.1 Documentation Practices
- •22.4.2 Considerations for Forensic Toxicological Analysis
- •22.4.3 Drug Concentrations and Distribution
- •22.5 Laboratory Analyses
- •22.5.1 Colorimetric Screening Tests
- •22.5.2 Thermal Desorption
- •22.5.6 Enzymatic Immunoassay
- •22.6 Analytical Schemes for Toxicant Detection
- •22.7 Clinical Toxicology
- •22.7.1 History Taking
- •22.7.2 Basic Operating Rules in the Treatment of Toxicosis
- •22.7.3 Approaches to Selected Toxicoses
- •Suggested Reading
- •23 Prevention of Toxicity
- •23.1 Introduction
- •23.2 Legislation and Regulation
- •23.2.1 Federal Government
- •23.2.2 State Governments
- •23.2.3 Legislation and Regulation in Other Countries
- •23.3 Prevention in Different Environments
- •23.3.1 Home
- •23.3.2 Workplace
- •23.3.3 Pollution of Air, Water, and Land
- •23.4 Education
- •Suggested Reading
- •24 Human Health Risk Assessment
- •24.1 Introduction
- •24.2 Risk Assessment Methods
- •24.2.2 Exposure Assessment
- •24.2.3 Dose Response and Risk Characterization
- •24.3 Noncancer Risk Assessment
- •24.3.1 Default Uncertainty and Modifying Factors
- •24.3.2 Derivation of Developmental Toxicant RfD
- •24.3.3 Determination of RfD and RfC of Naphthalene with the NOAEL Approach
- •24.3.4 Benchmark Dose Approach
- •24.3.5 Determination of BMD and BMDL for ETU
- •24.3.6 Quantifying Risk for Noncarcinogenic Effects: Hazard Quotient
- •24.3.7 Chemical Mixtures
- •24.4 Cancer Risk Assessment
- •24.5 PBPK Modeling
- •Suggested Reading
- •25 Analytical Methods in Toxicology
- •25.1 Introduction
- •25.2 Chemical and Physical Methods
- •25.2.1 Sampling
- •25.2.2 Experimental Studies
- •25.2.3 Forensic Studies
- •25.2.4 Sample Preparation
- •25.2.6 Spectroscopy
- •25.2.7 Other Analytical Methods
- •Suggested Reading
- •26 Basics of Environmental Toxicology
- •26.1 Introduction
- •26.2 Environmental Persistence
- •26.2.1 Abiotic Degradation
- •26.2.2 Biotic Degradation
- •26.2.3 Nondegradative Elimination Processes
- •26.3 Bioaccumulation
- •26.4 Toxicity
- •26.4.1 Acute Toxicity
- •26.4.2 Mechanisms of Acute Toxicity
- •26.4.3 Chronic Toxicity
- •26.4.5 Abiotic and Biotic Interactions
- •26.5 Conclusion
- •Suggested Reading
- •27.1 Introduction
- •27.2 Sources of Toxicants to the Environment
- •27.3 Transport Processes
- •27.3.1 Advection
- •27.3.2 Diffusion
- •27.4 Equilibrium Partitioning
- •27.5 Transformation Processes
- •27.5.1 Reversible Reactions
- •27.5.2 Irreversible Reactions
- •27.6 Environmental Fate Models
- •Suggested Reading
- •28 Environmental Risk Assessment
- •28.1 Introduction
- •28.2 Formulating the Problem
- •28.2.1 Selecting Assessment End Points
- •28.2.2 Developing Conceptual Models
- •28.2.3 Selecting Measures
- •28.3 Analyzing Exposure and Effects Information
- •28.3.1 Characterizing Exposure
- •28.3.2 Characterizing Ecological Effects
- •28.4 Characterizing Risk
- •28.4.1 Estimating Risk
- •28.4.2 Describing Risk
- •28.5 Managing Risk
- •Suggested Reading
- •29 Future Considerations for Environmental and Human Health
- •29.1 Introduction
- •29.2 Risk Management
- •29.3 Risk Assessment
- •29.4 Hazard and Exposure Assessment
- •29.5 In vivo Toxicity
- •29.6 In vitro Toxicity
- •29.7 Biochemical and Molecular Toxicology
- •29.8 Development of Selective Toxicants
- •Glossary
- •Index

166 CHEMICAL AND PHYSIOLOGICAL INFLUENCES ON XENOBIOTIC METABOLISM
|
|
|
|
Fe, Cu, Glycine, |
|
|
Protein, |
Niacin |
Protein |
Riboflavin |
Pantothenic acid, |
|
|
Pyridoxine |
|
|
||||
Glucose |
|
|
|
|
|
|
|
|
|
|
|
|
|
|
NADP |
|
Reduced |
|
|
|
|
|
FAD, FMN |
|
|
|
|
Glucose-6-P |
|
Cytochrome |
|
Reduced |
Oxidized |
ROH |
Dehydrogenase |
|
Reductase |
|
Cytochrome |
Cytochrome |
(Oxidized |
|
|
|
|
|
|
Substrate) |
|
NADPH |
|
Oxidized |
|
|
RH (Substrate) |
|
|
|
|
|
FAD, FMN
Nutritional Requirement
Figure 9.1 Nutritional requirements with potential effects on the cytochrome P450 monooxygenase system (From W. E. Donaldeson Nutritional factors, in Introduction to Biochemical Toxicology, 3rd ed., E. Hodgson and R. C. Smart, Wiley, 2001.)
could be made for other phase I reaction systems such as arachidonic acid cooxidations, the glutathione peroxidase system, and so on.
Phase II Reactions. As with phase I reactions, phase II reactions usually depend on several enzymes with different cofactors and different prosthetic groups and, frequently, different endogenous cosubstrates. All of these many components can depend on nutritional requirements, including vitamins, minerals, amino acids, and others. Mercapturic acid formation can be cited to illustrate the principles involved. The formation of mercapturic acids starts with the formation of glutathione conjugates, reactions catalyzed by the glutathione S-transferases.
This is followed by removal of the glutamic acid and the glycine residues, which is followed by acetylation of the remaining cysteine. Essential amino acids are required for the synthesis of the proteins involved, pantothenic acid for coenzyme A synthesis, and phosphorus for synthesis of the ATP needed for glutathione synthesis. Similar scenarios can be developed for glucuronide and sulfate formation, acetylation, and other phase II reaction systems.
9.3PHYSIOLOGICAL EFFECTS
9.3.1Development
Birth, in mammals, initiates an increase in the activity of many hepatic enzymes, including those involved in xenobiotic metabolism. The ability of the liver to carry out monooxygenation reactions appears to be very low during gestation and to increase after birth, with no obvious differences being seen between immature males and females. This general trend has been observed in many species, although the developmental pattern may vary according to gender and genetic strain. The component enzymes of the P450 monooxygenase system both follow the same general trend, although there
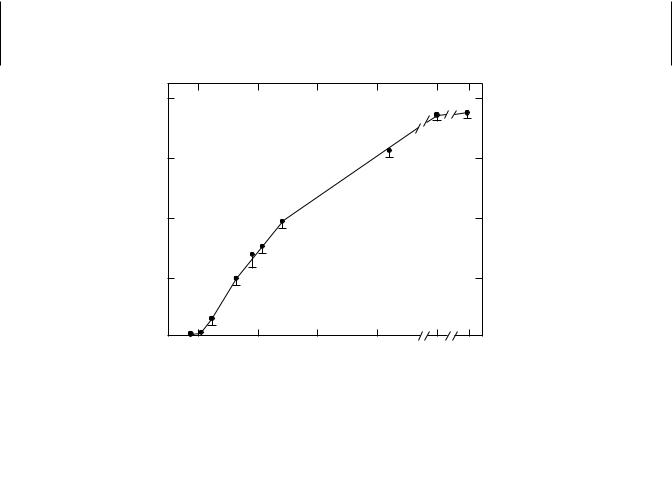
PHYSIOLOGICAL EFFECTS |
167 |
may be differences in the rate of increase. In the rabbit, the postnatal increase in P450 and its reductase is parallel; in the rat, the increase in the reductase is slower than that of the cytochrome.
Phase II reactions may also be age dependent. Glucuronidation of many substrates is low or undetectable in fetal tissues but increases with age. The inability of newborn mammals of many species to form glucuronides is associated with deficiencies in both glucuronosyltransferase and its cofactor, uridine diphosphate glucuronic acid (UDPGA). A combination of this deficiency, as well as slow excretion of the bilirubin conjugate formed, and the presence in the blood of pregnanediol, an inhibitor of glucuronidation, may lead to neonatal jaundice. Glycine conjugations are also low in the newborn, resulting from a lack of available glycine, an amino acid that reaches normal levels at about 30 days of age in the rat and 8 weeks in the human. Glutathione conjugation may also be impaired, as in fetal and neonatal guinea pigs, because of a deficiency of available glutathione. In the serum and liver of perinatal rats, glutathione transferase is barely detectable, increasing rapidly until all adult levels are reached at about 140 days (Figure 9.2). This pattern is not followed in all cases, because sulfate conjugation and acetylation appear to be fully functional and at adult levels in the guinea pig fetus. Thus some compounds that are glucuronidated in the adult can be acetylated or conjugated as sulfates in the young.
An understanding of how these effects may be related to the expression of individual isoforms is now beginning to emerge. It is known that in immature rats of either gender, P450s 2A1, 2D6, and 3A2 predominate, whereas in mature rats, the males show a predominance of P450s 2C11, 2C6, and 3A2 and the females P450s 2A1, 2C6, and 2C12.
The effect of senescence on the metabolism of xenobiotics has yielded variable results. In rats monooxygenase activity, which reaches a maximum at about 30 days
|
80 |
|
|
|
|
|
|
−1 |
60 |
|
|
|
|
|
|
· ml Serum |
|
|
|
|
|
|
|
|
|
|
|
|
|
|
|
−1 |
40 |
|
|
|
|
|
|
nmoles · min |
|
|
|
|
|
|
|
20 |
|
|
|
|
|
|
|
|
|
|
|
|
|
|
|
|
−10 |
0 |
20 |
40 |
60 |
140 |
180 |
Age (days)
Figure 9.2 Developmental pattern of serum glutathione S-transferase activity in female rats. (Adapted from H. Mukhtar and J. R. Bend, Life Sci. 21: 1277, 1977.)

168 CHEMICAL AND PHYSIOLOGICAL INFLUENCES ON XENOBIOTIC METABOLISM
of age, begins to decline some 250 days later, a decrease that may be associated with reduced levels of sex hormones. Glucuronidation also decreases in old animals, whereas monoamine oxidase activity increases. These changes in the monooxygenase activities are often reflected by changes in drug efficacy or overall toxicity.
In humans, age-related impairment of enzyme activity is highly controversial. Agerelated declines in activity were not detected with respect to the activity of CYP2C and CYP3A isoforms among 54 liver samples from donors ranging in age from 9 to 89 years. Studies involving an erythromycin breath test in humans also suggested that there were no age-related declines associated with CYP3A4 activity. However, a study of CYP content and antipyrine clearance in liver biopsies obtained from 226 closely matched subjects indicated that subjects older than 70 had significantly less activity and clearance than younger subjects. Likewise, in older subjects, clearance of the drug omeprazole, a CYP2C19 substrate, was nearly half the rates observed in younger subjects.
9.3.2Gender Differences
Metabolism of xenobiotics may vary with the gender of the organism. Gender differences become apparent at puberty and are usually maintained throughout adult life. Adult male rats metabolize many compounds at rates higher than females, for example, hexobarbital hydroxylation, aminopyrine N -demethylation, glucuronidation of o-aminophenol, and glutathione conjugation of aryl substrates; however, with other substrates, such as aniline and zoxazolamine, no gender differences are seen. In other species, including humans, the gender difference in xenobiotic metabolism is less pronounced. The differences in microsomal monooxygenase activity between males and females have been shown to be under the control of sex hormones, at least in some species. Some enzyme activities are decreased by castration in the male and administration of androgens to castrated males increases the activity of these sex-dependent enzyme activities without affecting the independent ones. Procaine hydrolysis is faster in male than female rats, and this compound is less toxic to the male. Gender differences in enzyme activity may also vary from tissue to tissue. Hepatic microsomes from adult male guinea pigs are less active in the conjugation of p-nitrophenol than are those from females, but no such gender difference is seen in the microsomes from lung, kidney, and small intestines.
Many differences in overall toxicity between males and females of various species are known (Table 9.1). Although it is not always known whether metabolism is the only or even the most important factor, such differences may be due to gender-related differences in metabolism. Hexobarbital is metabolized faster by male rats; thus female rats have longer sleeping times. Parathion is activated to the cholinesterase inhibitor paraoxon more rapidly in female than in male rats, and thus is more toxic to females. Presumably many of the gender-related differences, as with the developmental differences, are related to quantitative or qualitative differences in the isozymes of the xenobiotic-metabolizing enzymes that exist in multiple forms, but this aspect has not been investigated extensively.
In the rat, sexually dimorphic P450s appear to arise by programming, or imprinting, that occurs in neonatal development. This imprinting is brought about by a surge of testosterone that occurs in the male, but not the female, neonate and appears to imprint the developing hypothalamus so that in later development the growth hormone

PHYSIOLOGICAL EFFECTS |
169 |
Table 9.1 Gender-Related Differences in Toxicity
Species |
Toxicant |
Susceptibility |
|
|
|
|
|
Rat |
EPN, warfarin, strychnine, |
F |
> M |
|
hexobarbital, parathion |
|
|
|
Aldrin, lead, epinephrine, ergot |
M > F |
|
|
alkaloids |
|
|
Cat |
Dinitrophenol |
F |
> M |
Rabbit |
Benzene |
F |
> M |
Mouse |
Folic acid |
F |
> M |
|
Nicotine |
M > F |
|
Dog |
Digitoxin |
M > F |
|
|
|
|
|
is secreted in a gender-specific manner. Growth hormone production is pulsatile in adult males with peaks of production at approximately 3-hour intervals and more continuous in females, with smaller peaks. This pattern of growth hormone production and the higher level of circulating testosterone in the male maintain the expression of male-specific isoforms such as P450 2C11. The more continuous pattern of growth hormone secretion and the lack of circulating testosterone appears to be responsible for the expression of female specific isoforms such as P450 2C12. The high level of sulfotransferases in the female appears to be under similar control, raising the possibility that this is a general mechanism for the expression of gender-specific xenobiotic-metabolizing enzymes or their isoforms. A schematic version of this proposed mechanism is seen in Figure 9.3.
Gender-specific expression is also seen in the flavin-containing monooxygenases. In mouse liver FMO1 is higher in the female than in the male, and FMO3, present at high levels in female liver, is not expressed in male liver (Figure 9.4). No gender-specific differences are observed for FMO5. The important role of testosterone in the regulation of FMO1 and FMO3 was demonstrated in gonadectomized animals with and without testosterone implants. In males, castration increased FMO1 and FMO3 expression to levels similar to those observed in females, and testosterone replacement to castrated males resulted in ablation of FMO3 expression. Similarly, administration of testosterone to females caused ablation of FMO3 expression. Although these results clearly indicate a role for testosterone in the regulation of these isoforms, the physiological reasons for their gender-dependent expression remain unknown.
9.3.3Hormones
Hormones other than sex hormones are also known to affect the levels of xenobiotic metabolizing enzymes, but these effects are much less studied or understood.
Thyroid Hormone. Treatment of rats with thyroxin increases hepatic microsomal NADPH oxidation in both male and female rats, with the increase being greater in females. Cytochrome P450 content decreases in the male but not in the female. Hyperthyroidism causes a decrease in gender-dependent monooxygenase reactions and appears to interfere with the ability of androgens to increase the activity of the enzymes responsible. Gender differences are not seen in the response of mice and rabbits to

170 CHEMICAL AND PHYSIOLOGICAL INFLUENCES ON XENOBIOTIC METABOLISM
TESTES
Testosterone
HYPOTHALAMUS
GHRH
PITUITARY
GH Secretion
|
|
1 day |
1 day |
||
|
Adult Male |
Adult Female |
|||
|
|
Pattern |
Pattern |
||
|
|
|
|
|
|
|
|
|
|
|
|
|
|
LIVER |
|
|
|
|
|
|
|
||
|
|
|
|
|
|
|
|
|
|
||
Adult Male |
Adult Female |
||||
P450 2C11 |
P450 2C12 |
Figure 9.3 Hypothetical scheme for neonatal imprinting of the hypothalamus–pituitary–liver axis resulting in sexually dimorphic expression of hepatic enzymes in the adult rat. Neonatal surges of testosterone appear to play a role in imprinting. (From M. J. J. Ronis and H. C. Cunny, in Introduction to Biochemical Toxicology, 2nd ed. E. Hodgson and P. E. Levi, eds., Appleton and Lange, 1994, p. 136.)
A
FMO1
C |
1 |
2 |
3 |
4 |
5 |
6 |
7 |
8 |
9 |
B |
|
|
|
|
|
|
|
|
|
FMO3 |
|
|
|
|
|
|
|
|
--- FMO3 |
C |
1 |
2 |
3 |
4 |
5 |
6 |
7 |
8 |
9 |
C |
|
|
|
|
|
|
|
|
|
FMO5 |
|
|
|
|
|
|
|
|
|
C |
1 |
2 |
3 |
4 |
5 |
6 |
7 |
8 |
9 |
FMO Control |
Male |
Sham Male |
Castrate |
Castrate + Test. |
Female |
Female + Test. |
Ovex |
Ovex + Test. |
Sham Female |
Figure 9.4 Immunoreactivity of liver microsomes from sexually intact control, sham control, gonadectomized mice, or mice undergoing gonadectomy and/or receiving testosterone implants (5 mg). (From J. G. Falls et al., Arch. Biochem. Biophys. 342: 212–223, 1997.)
- #15.08.20134.04 Mб15Hastie T., Tibshirani R., Friedman J. - The Elements of Statistical Learning Data Mining, Inference and Prediction (2002)(en).djvu
- #
- #
- #
- #
- #
- #
- #
- #15.08.201315.44 Mб23Hudlicky M, Pavlath A.E. (eds.) - Chemistry of Organic Fluorine Compounds 2[c] A critical Review (1995)(en).djvu
- #
- #