
Garrett R.H., Grisham C.M. - Biochemistry (1999)(2nd ed.)(en)
.pdf
21.12 ● ATP Exits the Mitochondria via an ATP–ADP Translocase |
701 |
21.12 ● ATP Exits the Mitochondria via an
ATP–ADP Translocase
ATP, the cellular energy currency, must exit the mitochondria to carry energy throughout the cell, and ADP must be brought into the mitochondria for reprocessing. Neither of these processes occurs spontaneously because the highly charged ATP and ADP molecules do not readily cross biological membranes. Instead, these processes are mediated by a single transport system, the ATP– ADP translocase. This protein tightly couples the exit of ATP with the entry of ADP so that the mitochondrial nucleotide levels remain approximately constant. For each ATP transported out, one ADP is transported into the matrix. The translocase, which accounts for approximately 14% of the total mitochondrial membrane protein, is a homodimer of 30-kD subunits. Transport occurs via a single nucleotide-binding site, which alternately faces the matrix and the cytosol (Figure 21.32). It binds ATP on the matrix side, reorients to face the cytosol, and exchanges ATP for ADP, with subsequent movement back to the matrix face of the inner membrane.
Outward Movement of ATP Is Favored over Outward ADP Movement
The charge on ATP at pH 7.2 or so is about 4, and the charge on ADP at the same pH is about 3. Thus, net exchange of an ATP (out) for an ADP (in) results in the net movement of one negative charge from the matrix to the cytosol. (This process is equivalent to the movement of a proton from the cytosol to the matrix.) Recall that the inner membrane is positive outside (see Figure 21.22), and it becomes clear that outward movement of ATP is favored over outward ADP transport, ensuring that ATP will be transported out (Figure 21.32). Inward movement of ADP is favored over inward movement of ATP for the same reason. Thus, the membrane electrochemical potential itself controls the specificity of the ATP–ADP translocase. However, the electrochemical potential is diminished by the ATP–ADP translocase cycle and therefore operates with an energy cost to the cell. The cell must compensate by passing yet more electrons down the electron transport chain.
What is the cost of ATP–ADP exchange relative to the energy cost of ATP synthesis itself? We already noted that moving 1 ATP out and 1 ADP in is the equivalent of one proton moving from the cytosol to the matrix. Synthesis of an
Matrix – |
+ |
Cytosol |
– |
+ |
|
– |
|
|
ATP |
|
|
ADP3–
–+
+–
|
+ |
H+ |
1 ATP out for 1 ADPin = 1 H+ in (= 1 –chargeout) |
– +
–+
– +
FIGURE 21.32 ● Outward transport of ATP (via the ATP/ADP translocase) is favored by the membrane electrochemical potential.


704 Chapter 21 ● Electron Transport and Oxidative Phosphorylation
reform dihydroxyacetone phosphate and enzyme-bound FADH2. The two electrons of [FADH2] are passed directly to UQ , forming UQH2. Thus, via this shuttle, cytosolic NADH can be used to produce mitochondrial [FADH2] and, subsequently, UQH2. As a result, cytosolic NADH oxidized via this shuttle route yields only 1.5 molecules of ATP. The cell “pays” with a potential ATP molecule for the convenience of getting cytosolic NADH into the mitochondria. Although this may seem wasteful, there is an important payoff. The glycerophosphate shuttle is essentially irreversible, and even when NADH levels are very low relative to NAD , the cycle operates effectively.
The Malate–Aspartate Shuttle Is Reversible
The second electron shuttle system, called the malate–aspartate shuttle, is shown in Figure 21.34. Oxaloacetate is reduced in the cytosol, acquiring the electrons of NADH (which is oxidized to NAD ). Malate is transported across the inner membrane, where it is reoxidized by malate dehydrogenase, converting NAD to NADH in the matrix. This mitochondrial NADH readily enters the electron transport chain. The oxaloacetate produced in this reaction cannot cross the inner membrane and must be transaminated to form aspartate, which can be transported across the membrane to the cytosolic side. Transamination in the cytosol recycles aspartate back to oxaloacetate. In contrast to the glycerol phosphate shuttle, the malate–aspartate cycle is reversible, and it operates as shown in Figure 21.34 only if the NADH/NAD ratio in the cytosol is higher than the ratio in the matrix. Because this shuttle produces NADH in the matrix, the full 2.5 ATPs per NADH are recovered.
The Net Yield of ATP from Glucose Oxidation
Depends on the Shuttle Used
The complete route for the conversion of the metabolic energy of glucose to ATP has now been described, in Chapters 19 through 21. Assuming appropriate P/O ratios, the number of ATP molecules produced by the complete oxidation of a molecule of glucose can be estimated. Keeping in mind that P/O ratios must be viewed as approximate, for all the reasons previously cited, we will assume the values of 2.5 and 1.5 for the mitochondrial oxidation of NADH and succinate, respectively. In eukaryotic cells, the combined pathways of glycolysis, the TCA cycle, electron transport, and oxidative phosphorylation then yield a net of approximately 30 to 32 molecules of ATP per molecule of glucose oxidized, depending on the shuttle route employed (Table 21.4).
The net stoichiometric equation for the oxidation of glucose, using the glycerol phosphate shuttle, is
Glucose 6 O2 30 ADP 30 Pi 88n
6 CO2 30 ATP 36 H2O (21.32)
Because the 2 NADH formed in glycolysis are “transported” by the glycerol phosphate shuttle in this case, they each yield only 1.5 ATP, as already described. On the other hand, if these 2 NADH take part in the malate–aspartate shuttle, each yields 2.5 ATP, giving a total (in this case) of 32 ATP formed per glucose oxidized. Most of the ATP—26 out of 30 or 28 out of 32—is produced by oxidative phosphorylation; only 4 ATP molecules result from direct synthesis during glycolysis and the TCA cycle.
The situation in bacteria is somewhat different. Prokaryotic cells need not carry out ATP/ADP exchange. Thus, bacteria have the potential to produce approximately 38 ATP per glucose.

Table 21.4
Yield of ATP from Glucose Oxidation
Pathway |
ATP Yield |
|
|
per Glucose |
|
|
|
|
|
Glycerol– |
Malate– |
|
Phosphate |
Aspartate |
|
Shuttle |
Shuttle |
|
|
|
Glycolysis: glucose to pyruvate (cytosol) |
|
|
Phosphorylation of glucose |
1 |
1 |
Phosphorylation of fructose-6-phosphate |
1 |
1 |
Dephosphorylation of 2 molecules of 1,3-BPG |
2 |
2 |
Dephosphorylation of 2 molecules of PEP |
2 |
2 |
Oxidation of 2 molecules of glyceraldehyde-3- |
|
|
phosphate yields 2 NADH |
|
|
Pyruvate conversion to acetyl-CoA (mitochondria) |
|
|
2 NADH |
|
|
Citric acid cycle (mitochondria) |
|
|
2 molecules of GTP from 2 molecules |
|
|
of succinyl-CoA |
2 |
2 |
Oxidation of 2 molecules each of isocitrate, |
|
|
-ketoglutarate, and malate yields 6 NADH |
|
|
Oxidation of 2 molecules of succinate yields 2 [FADH2] |
|
|
Oxidative phosphorylation (mitochondria) |
|
|
2 NADH from glycolysis yield 1.5 ATP each if NADH |
|
|
is oxidized by glycerol-phosphate shuttle; 2.5 ATP |
|
|
by malate-aspartate shuttle |
3 |
5 |
Oxidative decarboxylation of 2 pyruvate to 2 acetyl-CoA: |
|
|
2 NADH produce 2.5 ATP each |
5 |
5 |
2 [FADH2] from each citric acid cycle produce |
|
|
1.5 ATP each |
3 |
3 |
6 NADH from citric acid cycle produce 2.5 ATP each |
15 |
15 |
Net Yield |
30 |
32 |
Note: These P/O ratios of 2.5 and 1.5 for mitochondrial oxidation of NADH and [FADH2] are “consensus values.” Because they may not reflect actual values and because these ratios may change depending on metabolic conditions, these estimates of ATP yield from glucose oxidation are approximate.
3.5 Billion Years of Evolution Have Resulted in
a System That Is 54% Efficient
Hypothetically speaking, how much energy does a eukaryotic cell extract from the glucose molecule? Taking a value of 50 kJ/mol for the hydrolysis of ATP under cellular conditions (Chapter 3), the production of 32 ATP per glucose oxidized yields 1600 kJ/mol of glucose. The cellular oxidation (combustion) of glucose yields G 2937 kJ/mol. We can calculate an efficiency for the pathways of glycolysis, the TCA cycle, electron transport, and oxidative phosphorylation of
1600 100% 54%
2937
This is the result of approximately 3.5 billion years of evolution.
705
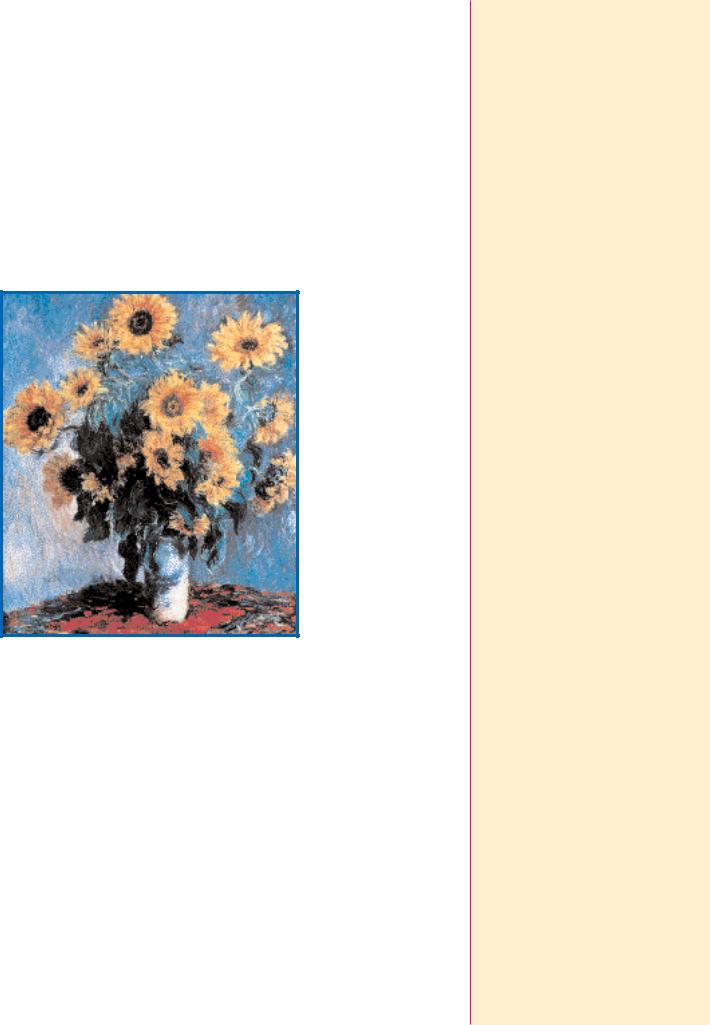
