
Metal-Catalysed Reactions of Hydrocarbons / 13-Reactions of the Lower Alkanes with Hydrogen
.pdf
REACTIONS OF THE LOWER ALKANES WITH HYDROGEN |
535 |
Figure 13.4. Compensation plot of Arrhenius parameters for hydrogenolysis of alkanes (C3 to C6 ) on Pt/SiO2 (EUROPT-1): circles, total rates; open triangles, hydrogenolysis only; filled triangles, isomerisation only; broken line shows location of points for ethane.33
TABLE 13.1. Apparent Activation Energies (kJ mol−1 ) for Hydrogenolysis (Eh ) and for Isomerisation (Ei ) of the Lower Alkanes
|
|
|
|
|
Eh |
|
|
|
Ei |
|
Metal |
Form |
C2 H6 |
C3 H8 |
n-C4 H10 |
iso-C4 H10 |
|
n-C4 H10 |
iso-C4 H10 |
References |
|
Ni |
film |
243 |
130 |
142 |
125 |
|
— |
— |
210 |
|
Ni |
/SiO2 |
191 |
167 |
128 |
153 |
|
— |
— |
89 |
|
Ru |
/TiO2 |
|
— |
137 |
133 |
118 |
|
— |
— |
100 |
Rh |
/TiO2 |
131 |
49 |
68 |
165 |
|
— |
— |
217 |
|
Rh |
/SiO2 |
|
— |
174 |
174 |
169 |
|
— |
— |
12 |
Rh |
/SiO2 |
|
— |
188 |
163 |
— |
|
— |
— |
47 |
Ir |
(111) |
145 |
140 |
132 |
152 |
|
— |
— |
8 |
|
Ir |
/SiO2 |
174 |
— |
174 |
243 |
|
— |
— |
9 |
|
Pt |
/A12 O3 |
219 |
150 |
135 |
161 |
105 |
116 |
17 |
||
Pt |
/A12 O3 |
|
— |
205 |
141 |
163 |
132 |
— |
43 |
|
Pt |
/SiO2 |
201 |
142 |
94 |
102 |
123 |
148 |
17 |
||
Pt |
/SiO2a |
199 |
189 |
129 |
— |
|
— |
— |
41 |
|
Pt |
black |
221 |
100 |
96 |
109 |
100 |
121 |
32 |
||
|
|
|
|
|
|
|
|
|
|
|
a EUROPT-1
Activation energies vary most with the least active metal (Pt).

536 |
CHAPTER 13 |
is not prefixed by the term ‘apparent’, as it should be, but the distinction between apparent and true values will be drawn where it is essential.
Orders of reaction using Power Rate Law formalism are rarely reported, but a few values are given in Table 13.2; temperatures of measurement are not always quoted.
TABLE 13.2. Kinetics of Hydrogenolysis of the Lower Alkanes
Metal |
Form |
Alkane |
nH |
nA |
T/K |
E/kJ mol−1 |
ln Ab |
References |
Fe |
Powder |
C2 H6 |
−0.7 |
1.0 |
500 |
— |
— |
207 |
Fe |
/SiO2 |
C2 H6 |
0.5 |
0.6 |
543 |
107 |
— |
24,25 |
Coa |
/SiO2 |
C2 H6 |
−0.8 |
1.0 |
492 |
125 |
58.7 |
24–27 |
Co |
/SiO2 |
C3 H8 |
−0.72 |
0.79 |
517 |
93 |
— |
37 |
Ni |
Powder |
C2 H6 |
−0.7 |
0.9 |
523 |
163 |
— |
60 |
Ni |
/SiO2 |
C2 H6 |
−2.4 |
1.0 |
450 |
170 |
73.0 |
24–27 |
Nia |
/SiO2 -A12 O3 |
C2 H6 |
−1.8 |
0.94 |
493 |
167 |
— |
20 |
Ni |
/SiO2 |
C3 H8 |
−1.57 |
0.73 |
528 |
175 |
— |
37 |
Ni |
/SiO2 model |
n-C4 H10 |
−2.2 |
0.8 |
473 |
113 |
— |
107 |
Ni |
/SiO2 -A12 O3 |
iso-C4 H10 |
−2.8 |
1.0 |
493 |
218 |
— |
20 |
Cu |
/SiO2 |
C2 H6 |
−0.4 |
1.0 |
580 |
89.5 |
47.5 |
27 |
Ru |
Black |
C2 H6 |
−0.1 |
0.92 |
456 |
119 |
59.0 |
38c |
Ru |
Sponge |
C2 H6 |
−1.10 |
1.03 |
461 |
88 |
51.8 |
206 |
Ru |
/A12 O3 |
C2 H6 |
−2.37 |
0.85 |
479 |
155 |
68.8 |
206 |
Ru |
/SiO2 |
C2 H6 |
−2.21 |
0.66 |
433 |
125 |
63.3 |
206 |
Ru |
/MgO |
C2 H6 |
−0.73 |
0.98 |
462 |
88 |
51.1 |
206 |
Rua |
Sponge |
C3 H8 |
−0.79 |
0.80 |
427 |
88 |
54.3 |
206 |
Ru |
/A12 O3 |
C3 H8 |
−2.07 |
0.54 |
409 |
120 |
— |
204 |
Ru |
/A12 O3 |
C3 H8 |
−1.98 |
0.80 |
440 |
155 |
— |
37 |
Ru |
/A12 O3 |
C3 H6 |
−0.9 |
0.65 |
464 |
142 |
68.3 |
206 |
Ru |
/SiO2 |
C3 H8 |
−1.3 |
0.65 |
416 |
113 |
64.4 |
206 |
Ru |
/MgO |
C3 H8 |
−0.82 |
0.92 |
427 |
75 |
50.1 |
206 |
Ru |
Black |
n-C4 H10 |
+0.3 |
0.81 |
443 |
— |
— |
38 |
Ru |
/A12 O3 |
iso-C4 H10 |
−0.66 |
0.74 |
373 |
151 |
— |
97 |
Rh |
Black |
C2 H6 |
−2.2 |
0.82 |
400 |
150 |
71.2 |
38 |
Rh |
/SiO2 |
C2 H6 |
−2.2 |
0.8 |
487 |
176 |
73.1 |
24 |
Rh |
Black |
n-C4 H10 |
−1.38 |
0.45 |
444 |
123 |
66.6 |
28 |
Pd |
/SiO2 |
C2 H6 |
−2.5 |
0.9 |
627 |
243 |
77.3 |
24 |
Os |
/SiO2 |
C2 H6 |
−1.2 |
0.6 |
425 |
146 |
71.0 |
24 |
Ir |
Black |
C2 H6 |
−1.65 |
0.72 |
444 |
167 |
65.5 |
38 |
Ir |
/A12 O3 |
C2 H6 |
−2.9 |
1.0 |
? |
174 |
67.4 |
9 |
Ir |
/SiO2 |
C2 H6 |
−1.6 |
0.7 |
483 |
151 |
66.1 |
24 |
Ir |
Black |
n-C4 H10 |
−0.3 |
0.42 |
468 |
100 |
52.6 |
38 |
Pt |
Black |
C2 H6 |
−1.9 |
0.9 |
690 |
221 |
62.0 |
38 |
Pt |
/A12 O3 |
C2 H6 |
−2.2 |
1.0 |
633 |
259 |
76.7 |
211 |
Pt |
/SiO2 |
C2 H6 |
−1.5 |
0.95 |
630 |
199 |
68.3 |
41 |
a Values of kinetic parameters depend on operating conditions. b A in molecules cm−2 s−1 (assuming 1015 sites cm−2 ).
c This paper gives results for other Ru loadings and supports, and, for C3 H8 , orders as a function of temperature (for Ru/MgO and Ru black).

REACTIONS OF THE LOWER ALKANES WITH HYDROGEN |
537 |
Figure 13.5. As Figure 13.3 but for cobalt, nickel and copper catalysts. See footnote to Fig. 13.3; 1 = Ni, 2 = Co, 3 = Cu.
We may consider results for other metals in the same way. Figure 13.5 shows Arrhenius parameters for cobalt, nickel and copper; most of them are for nickel.20 Many of them lie inside a quite narrow band, but a number of those for ethane (filled points) lie well below it, as do those for higher alkanes. The reasons for these discrepancies are not immediately clear: there are few causes for a too high specific rate being recorded (under-estimation of the active area is one), but several for a too low rate (incomplete reduction, especially of the base metals, if areas are based on TEM, and particularly loss of active area early in use due to ‘carbon’ deposition). Results for ruthenium catalysts are displayed in Figure 13.6; a good compensation plot is again seen, with the values for ethane lying close to the bottom of the band. Quite a large number of results are available for rhodium catalysts (Figure 13.7); the points for ethane now lie about a distinctly separate lower line. Results for iridium catalysts are shown in Figure 13.8; parameters for ethane are now contained within the band, only a few points for metal blacks lying below it. A selection of the Power Rate Law orders with the associated Arrhenius parameters is contained within Table 13.2.
This broader survey of rates and Arrhenius parameters should harmonise with and perhaps extend the generalisation contained in Figure 5.15, which was based only on ethane, and this as we have seen tends to be somewhat less reactive than the larger alkanes. From the central lines of Figures 13.3 and 13.5–13.8 values of ln A at E = 175 kJ mol−1 are interpolated, and with values of isokinetic temperatures Ti are compared in Table 13.3. The agreement is fair (except for Ti in the case of iridium), so we may conclude that this wider comparison generally supports the earlier classification.

538 |
CHAPTER 13 |
Figure 13.6. As Figure 13.3 but for ruthenium and osmium catalysts. See footnote to fig. 13.3; 1 = Ru; 2 = Os.
The Arrhenius parameters forming the substance of Figures 13.3 and 13.5–13.8 and Table 13.1 have naturally been obtained under a variety of conditions, especially of reactant pressures (which are sometimes not noted). It is surprising to say the least that, irrespective of the numbers and ranges of the variables involved, the values of the Arrhenius parameters form such a tightly grouped set. It is now necessary to introduce orders of reaction into the discussion. These are rarely measured at more than a single temperature; with Sinfelt’s data set for ethane
Figure 13.7. As Figure 13.3 but for rhodium catalysts. See footnote to Fig. 13.3.

REACTIONS OF THE LOWER ALKANES WITH HYDROGEN |
539 |
Figure 13.8. As for Figure 13.3 but for iridium catalysts. See footnote to Fig. 13.3.
hydrogenolysis we have already noted a trend of hydrogen order to become more positive as the temperature of measurement was raised, but since different metals were used the conclusion is unsafe. An extensive study36 of a number of nickel catalysts has shown that the rates of propane hydrogenolysis to ethane + methane, and methane only, generate Arrhenius parameters that show excellent compensation, and the activation energies show a marked correlation with hydrogen orders (order, -3; E = 270 kJ mol−1: order, zero; E = 55 kJ mol−1 ). Perhaps the best confirmation of the connection in the older literature is provided by hydrogen orders for propane hydrogenolysis measured at three temperatures on four catalysts;37 in each case the order rose smoothly, and the value at 573 K was a linear function of activation energy (see Figure 13.9). There is clearly an intimate connection between hydrogen order and activation energy. There have also been suggestions that the value of the activation energy depends on the hydrogen pressure used to measure it,38−40 and the feeling therefore arose that, far from being divinely decided to characterise a metal, it was in fact a very moveable feast. A further trend that is sometimes apparent is for the hydrogen order to become less negative, and that for the alkane to become less positive, as alkane chain length increases, but on the whole the alkane order is relatively insensitive to variations in catalyst structure and composition, to alkane chain length, and to experimental conditions.9,32,41,42
On iridium9 and platinum43 catalysts, the activation energies for isobutane hydrogenolysis were always higher than that for n-butane, reflecting perhaps the

540 CHAPTER 13
TABLE 13.3. Comparison of Coordinates of Compensation Lines from the Results of J.H. Sinfelt and those in Figures 13.3 and 13.5–13.8
|
Isokinetic Temperature/K |
Value of ln A at E = 175 |
|
|||
Metal(s) |
JHS |
Figures |
|
JHS |
Figures |
|
|
|
|
|
|
|
|
Ni, Co |
401 |
316 |
|
73 |
77 |
|
Ru |
340 |
353 |
|
80 |
80 |
|
Rh |
401 |
445 |
|
73 |
77 |
|
Ir |
401 |
650 |
|
73 |
72.7 |
|
Pt, Pd |
650 |
600 |
|
64 |
62.5 |
|
|
|
|
|
|
|
|
impossibility of forming more than a single C M bond at the tertiary carbon atom. However, this distinction is not consistently shown by other metals (Table 13.1), and arguments based purely on activation energy have to be used with care,9 especially where compensation occurs (see Figure 13.8).
13.2.3. Mechanisms and Kinetic Formulations
Interpretation of the work of Sir Hugh Taylor and his associates at Princeton was based on the following mechanism:
2 |
|
6 ←−−→ |
2 |
x + |
|
2 |
+ |
|
2 |
|
2 |
|
C |
H |
K1 |
C |
H |
H |
|
|
6 |
− x |
H |
|
(13.A) |
|
|
|
|
|
||||||||
|
|
|
|
|
|
|||||||
C2Hx + H2 −→ CHp |
+ CHq |
|
|
(13.B) |
Figure 13.9. Dependence on temperature of hydrogen orders for hydrogenolysis of propane on iron (4), cobalt (3) and nickel (1 and 2) catalysts.37
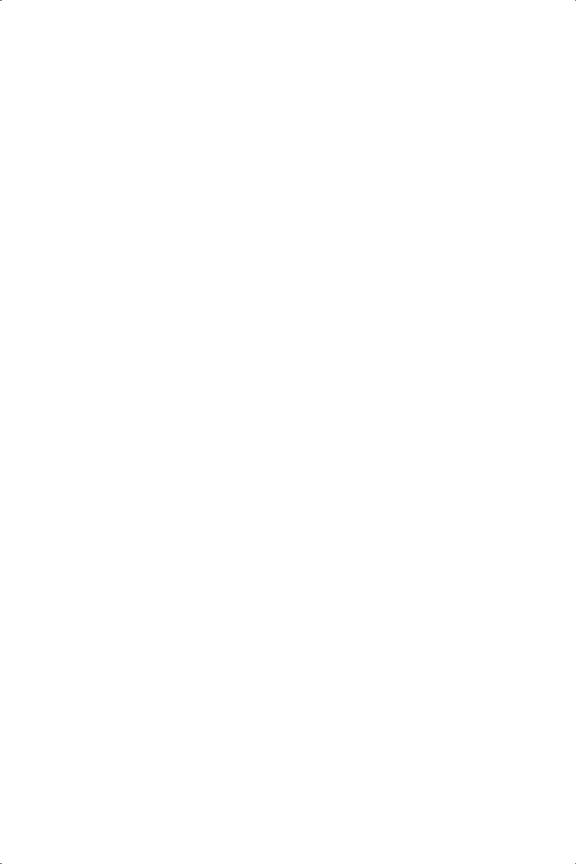
REACTIONS OF THE LOWER ALKANES WITH HYDROGEN |
541 |
Equilibrium was assumed to be maintained between ethane, C2Hx and gaseous hydrogen, and the mono-carbon species were swiftly somehow converted into methane: the second process was rate-determining. This led to a rate expression of the form
r = k PEy PH(1−ya) |
(13.5) |
where a is (6 − x)/2. John Sinfelt subsequently extended 24,25 this analysis to the following reaction scheme:
2 |
|
k1 |
2 |
5 |
+ |
|
K2 |
2 |
x |
+ |
|
2 |
|
2 |
|
|
|
6 −→ |
|
−→ |
|
|
|||||||||||
C |
H |
←− |
C |
H |
|
H |
←− |
C |
H |
6 |
− x |
H |
|
(13.C) |
||
|
|
|
|
|
||||||||||||
|
|
|
|
|
|
|
|
|
|
|
|
|
|
|
||
|
|
k−1 |
|
|
k3 |
|
|
|
|
|
|
|
|
|
|
|
|
C2Hx |
|
|
|
|
|
|
|
|
|
|
|
|
(13.D) |
||
|
+ H2 −→ CHp + CH2 |
|
|
|
|
|
||||||||||
which gives the rate expression |
|
|
|
|
|
|
|
|
|
|
|
|
||||
|
|
|
r = k1 PE/(1 + b PH(a−1)) |
|
|
|
(13.6) |
|||||||||
where a as before is (6 − x)/2, and b is k1 /k3 K2. C |
|
C bond splitting was again taken |
||||||||||||||
|
to be rate-limiting. Measurement of the hydrogen orders for ethane hydrogenolysis over Co/SiO226 had shown that b decreased with increasing temperature, perhaps chiefly because the value of K2 became greater. Application of this analysis to his results for this reaction on a variety of metals led to estimates24,25 of the values of x,which ranged from zero for rhodium, palladium and platinum, two for nickel, ruthenium, osmium and iridium, and four for cobalt: answers could not be obtained for iron or rhenium, which had shown positive orders in hydrogen. It was accepted that there was no competition between ethane and hydrogen for surface sites, so there was no provision for the independent chemisorption of hydrogen, and no detailed site counting, the single asterisk simply indicating an adsorbed state. Despite its limitations, the procedure was widely adopted by other scientists,44 and has been extended.45
Subsequent developments have addressed these and other deficiencies, which give rise to the following questions.
What is the site requirement for the initial chemisorption of the alkane, and for each of the subsequent steps? How does this vary with the size of the alkane?
What is the mechanism by which the C2 species disintegrates?
Does hydrogen chemisorb in competition with the alkane?

542 |
CHAPTER 13 |
Are hydrogen atoms or only hydrogen molecules involved?
Which is the rate-determining step?
In addressing these questions, the following considerations must be kept in mind. (1) The behaviour of propane and especially the butanes differs significantly from that of ethane; activation energies are lower, orders in the alkane are often fractionally positive and hydrogen orders are sometimes less negative. (2) The Power Rate Law formalism and the derivation of reaction orders as exponents of the reactant pressures overlooks the fact that the rate must become zero and not infinite when no hydrogen is present, and must therefore pass through a maximum as its pressure is changed. The unfortunate habit of showing results as log-log plots4,16,46,47 disguises trends in the sense of dependence of rate on hydrogen pressure, although their non-linearity has often been noted, and maxima have sometimes been detected.16,20 What is even more regrettable is the reporting of the dependence of rates upon experimental variables only in terms of some cherished theoretical framework, so that their reinterpretation by some other model becomes a virtual impossibility.
Leclercq and her colleagues10,12 have made much use of an equation based on the mechanisms shown above, in which the alkane adsorbs by losing hydrogen atoms in pairs (not singly); they immediately combine to form a gaseous molecule (process 13.A), this needing only a single site to accommodate the dehydrogenated species. Introduction of the independent chemisorption of hydrogen and detailed consideration of the relevant form of isotherm led with some simplifications to the expression
r = k3 K PC PH/(K PC + PHa + mx ) |
(13.7) |
where K = K1/bH, m is the size of the ensemble on which reaction takes place and x is defined as
(1 + (bHPH)1/2 ) ≈ (bHPH)x |
|
|
(13.8) |
||
Linearisation of this equation has allowed evaluation of k3, K |
and (a |
+ |
mx) |
||
for the hydrogenolysis of a number of alkanes on nickel |
12,20,48 |
|
|
49 |
|
|
and platinum |
|
catalysts (also Ir/Al2O3; results for alkanes with more than four carbon atoms will be mentioned in the next chapter.
Opposing views have been expressed concerning the location of the ratedetermining step. Martin and his associates50−52 have taken this to be the initial chemisorption of the alkane, which they believed to require an ensemble of m atoms, similar in size to that which their magnetic studies had suggested. Careful work on EUROPT-151 involving hydrogen chemisorption, transient and

REACTIONS OF THE LOWER ALKANES WITH HYDROGEN |
543 |
steady-state kinetics led them to the rate expression |
|
r = k PE(1 − βθH)m |
(13.9) |
where β (= 1.32) represents the maximum value of H/Pts: thus the slow step is the collision of an ethane molecule with a group of m platinum atoms devoid of hydrogen atoms. The methodology of their further procedures is somewhat hard to follow, as they reported values of activation energy as a function of temperature and of hydrogen orders as a function of hydrogen pressure. On a PtFe/SiO2 catalyst it has however been shown51 that the hydrogen order becomes less negative and the n-butane order less positive as the hydrogen coverage increases. Use of the Temkin equation (5.29) with a heat of hydrogen chemisorption of 84 kJ mol−1 gave a true activation energy of 55 kJ mol−1 and a pre-exponential factor that was not much less than the estimated collision number for ethane. The value of m was nine; but similar work21 with propane hydrogenolysis on Ni/SiO2 gave values of m of 17 for single bond breaking and 24 for the breaking of both bonds. These numbers would seem to be on the generous side.
Equally thoughtful work by Frennet and his colleagues has led to the definite conclusion that the formation of methane from C1 fragments is ratedetermining.53−55 This conclusion was based on a comparison between rates of hydrogenolysis and of alkane exchange. Rates of ethane exchange were much the slower, but for many metals the rates of methane were comparable. Only for the base metals were they much slower, and for platinum much faster. This idea would require C1 species to be the MASI (Section 5.3). A thorough review56 to 1982 of proposed mechanisms wisely concludes that unequivocal identification of the slow step based on kinetic measurements alone is not possible. Clearly these theories cannot be simultaneously true; if one were to take the average, the slow step would be somewhere in the middle.
Speaking of which brings us to the excellent work of Dumesic and his associates, who have sought to apply the techniques of kinetic simulation to ethane hydrogenolysis, using measured or calculated values of H M and C M bond strengths.46,57−59 This approach, directed to Sinfelt’s kinetic measurements, brought them to the conclusion that on silica-supported platinum, palladium, iridium and cobalt the slow step is irreversible C C bond rupture in C2Hx (x = 4 or 3) and that hydrogenation of C1 species is kinetically insignificant. In a further contribution,46 new kinetic work on Pt/SiO2, backed by DFT calculations, permitted speculation as to the nature of the C C bond-breaking step: this was thought to be the spontaneous disruption of ethylidene (CH3CH ) by interaction with two vacant sites, and kinetic modelling based on this belief seemed to accord with experimental results obtained over a wide range of conditions (e.g. T = 573–673 K). Thus in a sense the wheel has come full circle; and having read these papers one wonders if there is anything left to be said on ethane hydrogenolysis.

544 |
CHAPTER 13 |
There are however a number of other points deserving comment. It is not certain that there is only one value for x in the reactive intermediate C2Hx, i.e. there may be several species differing in their reactivity; it was suggested some years ago that the composition of C2Hx might depend on the reactant ratio.40,60 A more catholic approach to deciding what mechanism best fits experimental results was adopted by Kristyan and his associates:61,62 writing the reaction set as
H2 |
+ |
|
bH |
|
|
|
|
|
|
|
(13.E) |
|
|
←− |
|
|
|
|
|
|
|
||||
|
2 −→ 2H |
|
|
|
|
|
|
|||||
C2H6 |
|
(7 |
|
x) |
KA |
|
|
(6 |
|
x)H |
(13.F) |
|
+ |
− |
−→ C2Hx |
+ |
− |
||||||||
|
|
|
|
←− |
|
|
|
|
||||
|
|
|
|
|
k |
|
|
|
|
|
|
|
C2Hx + B −→ CHp + CH2 |
|
|
|
(13.G) |
The identity of B was then taken as either or a hydrogen atom or a hydrogen molecule. This gave the alternative rate expressions shown in Table 13.4. Their own (somewhat unsatisfactory) results for ethane hydrogenolysis on nickel and palladium powders62 were best fitted by taking B to be a hydrogen molecule. Plus ca¸ change. Shang and Kenney63 pursued this broader approach and considered no less than ten rate expressions (there are unfortunately several errors in the equations in their Table 2). Their experimental work on ethane hydrogenolysis over Ru/SiO2 showed that the position of the rate maximum as hydrogen pressure was changed increased with ethane pressure and with temperature, and that the rate versus ethane pressure also exhibited a gentle maximum at a hydrogen pressure of 0.027 atm. Their analysis concluded that B = offered the best option, while admitting that discrimination between alternative rate expressions was not easy. This has already been demonstrated in Figure 5.9, where three alternative equations were applied to results for n-butane hydrogenolysis on a PtRe/A12O3 catalyst: two of them give virtually indistinguishable curves.
TABLE 13.4. Rate Expressions Based on the
Mechanism Given by Processes 13.E, F and G64
B |
r |
Code |
|
kyG6 /D2 |
ES5A |
H |
kyG7 /D2 |
ES5B |
H2 |
kyPH /D |
ES5 |
Definitions:
+ 1 y = KA PA ; G6 = (bH PH )a ; G7 = (bH PH )a /2
D = y + G6 + G7 ; a = (n − x)/2 where n is the number of carbon atoms in the alkane, and x the number of H atoms in the reactive species.