
Metal-Catalysed Reactions of Hydrocarbons / 13-Reactions of the Lower Alkanes with Hydrogen
.pdf
REACTIONS OF THE LOWER ALKANES WITH HYDROGEN |
575 |
good work and the separated oxides cannot on reduction re-form the bimetal.108 The combination of two metals into a bimetallic particle should be possible where mutual solubility in the bulk is extensive or complete.109 In such cases the main concern is whether preferential segregation of the component of lower surface energy to the surface occurs, and how it is distributed over sites that differ in coordination number.45,155,158 Such considerations initiate attempts159 to extract the size of the active ensemble from the dependence of rate upon composition based on simple statistics. We may however expect to see variations in product selectivity with composition where each component reaction has its specific site requirement.159,160
The discovery161,162 that bulk mutual solubility is not an essential pre-requisite for the formation of a stable surface bimetallic phase opened the way for extensive research, particularly on the ruthenium-copper system. The platinum-rhenium system has also proved of interest because of its use in petroleum reforming, but the platinum-iridium system, which is of comparable importance, has not been so widely examined with the smaller alkanes.163 It is convenient to classify the material to be considered in the following way.
(1)(A) Metals of Groups 10 and 11;
(B)metals of Groups 9 and 11;
(C)metals of Groups 8 and 11.
(2)Metals of Groups 8 to 10 and Groups 13 to 14.
(3)Metals of Groups 8 to 10 and Groups 4 to 7.
(4)Metal pairs formed within Groups 8 to 10.
Emphasis will be placed on those studies that illuminate reaction mechanisms.
13.8.2. Metals of Groups 8 to 10 plus Group 11
The early and systematic work by John Sinfelt and his associates on ethane hydrogenolysis using nickel-copper powders showed 24,164 that specific rates at 589 K fell catastrophically as copper content was increased, that at 74% copper being only 10−5 that of nickel. Together with the lack of dependence of the rate of cyclohexane dehydrogenation on composition, these results—perhaps the most often cited if not the best understood in bimetallic catalysis—were influential in distinguishing structure-sensitive reactions from the structure-insensitive. Over most of the composition range, excluding pure nickel, activation energies were constant, and orders of reaction changed slightly in the sense expected because of the necessary increase in temperature needed to determine them, because of the activity decrease. Rates therefore depended on the pre-exponential term (Figure 13.22) and hence on the number of active centres, the composition of which was uniform,

576 |
CHAPTER 13 |
Figure 13.22. Hydrogenolysis of ethane on nickel-copper powders: ln ( A/molecules cm−2 s−1 ) versus composition.164
there being no obvious ‘electronic factor’. The amount of hydrogen chemisorbed under ambient conditions was almost independent of composition, suggesting that Sachtler’s ‘cherry’ model was applicable; this predicted a constant surface composition between about 3 and 80% copper. However phase separation is only thermodynamically possible below about 470 K, so that at reaction temperatures (>600 K) homogeneous bimetallic particles were probably formed. In that case the rate would have depended on the chance of finding ensembles of the required size,45 and the observed effect would be qualitatively understood. Similar but less orderly results were obtained by John Clarke.165 There have been no reports of the use of nickel-silver or nickel-gold for reactions of the smaller alkanes.
With NiCu/SiO2 catalysts, rates of ethane hydrogenolysis declined with increasing copper content less fast than with the powders because the particles were smaller, but the kinetic parameters were similar.159 Experiments with propane and n-butane159,160 produced only one surprise: with the former, the ethane selectivity S2 rose appreciably as (0.45 to 0.85) the copper content was increased from zero to 35%. This favouring of reactive desorption, which as we have seen needs more hydrogen atoms for its completion than the further bond-breaking, cannot be due to restricted site size, but must be a consequence of a weakening of the C M bond strength. This is the only indication of any electronic interaction between the components.
In the reaction of n-butane over (111)-oriented palladium-copper and -silver films,166 the isomerisation selectivity jumped from about 10% to much higher values (25–75%) for the bimetallics, while the ethane selectivity was lowered, but rose again for copper contents above 50%. The use of various temperatures to accommodate the activity changes complicates interpretation. Deposition of gold onto

REACTIONS OF THE LOWER ALKANES WITH HYDROGEN |
577 |
platinum foil caused the rate of isobutane isomerisation at 573 K to decrease less quickly than its hydrogenolysis,167 while with graphite-supported platinum-gold particles derived from colloidal suspensions168,169 the rate of n-butane dehydrogenation at 633 K increased with gold content, but rates of isomerisation and (a fortiori) hydrogenolysis decreased. Temperature-independent product selectivities were only slightly changed by the inclusion of gold. Addition of silver to Rh/TiO2138 caused the rate of ethane hydrogenolysis at 673 K to decrease by 104, but a PtAu/SiO2 catalyst had about the same activity for isobutane hydrogenolysis as Pt/SiO2.170
The behaviour of the ruthenium-copper system has been widely studied (the osmium-copper system less so, but it was similar in all respects161). The effects are due to the extent to which copper atoms cover the surface of the ruthenium particles; thus with ruthenium powder of low dispersion a trace of copper (1.5%) had a dramatic effect,162 more than halving hydrogen uptake and lowering the specific rate of ethane hydrogenolysis by 103. With small silica-supported ruthenium particles,108,156,157,161 the same effect has only been found at a ruthenium/copper ratio of about unity. Mild oxidation (623 K) destroyed the bimetallic particles, and reduction at 433 K gave rates (for n-butane hydrogenolysis) characteristic of ruthenium, but a second reduction at 623 K gave larger metal particles, the activity of which fell faster with increasing copper content than did that of the original smaller ( 1 nm) particles.171 The choice of support is also important: most studies have used silica (see Further Reading section), and hydrogenolysis rates have usually fallen logarithmically with increasing copper content, but with alumina and magnesia minima have been seen,172 possibly due to the effect of support impurities. Hydrogenolysis of ethane took place without loss of activity,156 although with n-butane some deactivation occurred.155
Although temperature effects have been studied, results have often been presented as compensation plots171 or graphically155,156.161 or as plots of TOF versus copper content at several temperatures,156 so that precise information on Arrhenius parameters is hard to come by. The activation energy for ethane hydrogenolysis decreased from 134 to 105 kJ mol−1 with increasing copper content,162 and then stayed the same, in consequence of which TOFs became larger with bimetallic catalysts than with pure ruthenium: this may be a result of lesser ‘carbon’ deposition. However, in another work, activation energy increased from 138 to 184 kJ mol−1 with increasing copper content.173 The mechanism of the reaction on RuCu/SiO2 has been derived using isotopic transient methodology.174 A compensation plot containing a number of Arrhenius parameters for n-butane hydrogenolysis exhibited two distinct bands, the upper containing those for ruthenium alone and the lower those for bimetallic catalysts.156 This suggests that copper has some effect that is additional to lowering the number of ruthenium ensembles of the necessary size, such as a mild electronic modification of the active centre. This view is supported by the finding that with Ru82Cu18/SiO2 (and with Ru60Ag40/SiO2) the order

578 CHAPTER 13
TABLE 13.15. |
Hydrogenolysis of n-Butane on Ru/A12 O3 and RuGe/A12 O3 Catalysts |
||||||
|
|
Variously Pretreated79 |
|
|
|
||
%Ru |
%Ge |
Pretreatment |
r a |
S2 |
S3 |
F |
T3 |
1 |
0 |
HTR1 |
307 |
1.39 |
0.28 |
0.72 |
1.00 |
1 |
0.7 |
HTR1 |
42 |
1.05 |
0.37 |
0.50 |
0.74 |
4 |
0 |
HTR1 |
895 |
0.87 |
0.85 |
0.36 |
0.56 |
4 |
0.4 |
HTR1 |
70 |
0.76 |
0.39 |
0.26 |
0.53 |
1 |
0 |
O/LTR |
1216 |
0.71 |
0.43 |
0.14 |
0.50 |
1 |
0.7 |
O/LTR |
3454 |
0.71 |
0.38 |
0.15 |
0.45 |
1 |
0 |
HTR2 |
313 |
0.81 |
0.39 |
0.20 |
0.49 |
1 |
0.7 |
HTR2 |
28 |
0.83 |
0.31 |
0.16 |
0.37 |
|
|
|
|
|
|
|
|
a In mmol gRu |
−1 h−1 . |
|
|
|
|
|
|
in hydrogen at 508 K became distinctly more negative (−2.3 compared to −1.4), which signifies a stronger hydrogen chemisorption. Sensitivity to copper content appeared to vary somewhat with the alkane, being particularly large for isobutane,108 the Arrhenius parameters for which lay on a separate compensation line.
In the reaction of n-butane,155,171 product selectivities were not much changed by the introduction of copper, high values of S2 (1.05–1.20) being found at 453– 473 K: values of F were 0.5 to 0.6 and of T3 0.75 to 1.0. In one case, values of S3 were reported to exceed those of S1; this, while impossible, was not commented on. The same occurrence with platinum catalysts under some conditions has been ascribed to a preferential incorporation of C1 units into the ‘carbon’ deposit.
Similar effects have been obtained by adding either silver or gold to but these systems have not been widely investigated.
13.8.3. Metals of Groups 8 to 10 plus Groups 13 or 14
A thorough investigation has been reported of the effects of Group 14 (Ge, Sn, Pb) additives to Ru/Al2O3 catalysts made in various ways.79 Prior indications were that germanium might be randomly dispersed over the ruthenium, thus lowering ensemble size, while tin and lead might decorate edge sites selectively and hence simulate large particle behaviour. However, for this concept to succeed, it is necessary to start with ruthenium particles that are sufficiently large for these possibilities to develop. With very highly dispersed Ru/Al2O3 (Ru1; 12 atoms per particle, see Section 13.6), germanium had little effect except to lower activity until a Ge/Ru ratio of 0.31 was reached (Table 13.15). EXAFS showed that the germanium was in a positive oxidation state,116 so the effect resembled an SMSI more than bimetal formation. Perhaps the smallest particles became encapsulated leaving a few larger particles free, as the values of the selectivity parameters moved towards those of Ru3 (H/Ru = 0.25) (Table 13.15). Adding germanium to Ru3 caused further movement in the same direction; putting tin on Ru1 decreased activity
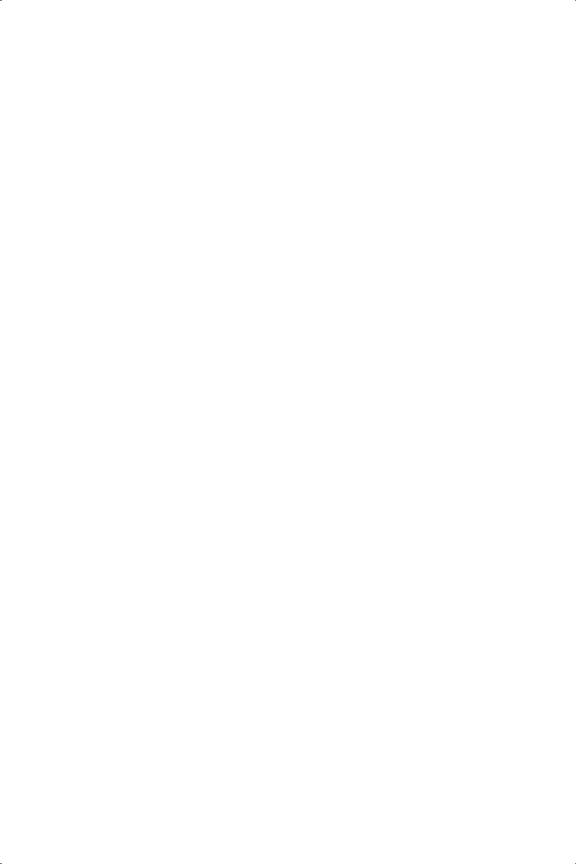
REACTIONS OF THE LOWER ALKANES WITH HYDROGEN |
579 |
markedly, but lead had little effect and neither altered selectivities noticeable. Oxidation (O/LTR) disassembled the components, and in the larger particles thus produced116 (Section 13.6) germanium was beneficial; a second reduction (HTR2) partially restored the connection. Determination of hydrogen orders and their interpretation by the ES5B equation (Table 13.4) showed that the effects produced by germanium on both catalysts were due to a decrease in bH and to a greater decrease in KA.
The beneficial effects of admixing tin with platinum for alkane dehydrogenation were noted in Section 12.23; the selectively-lowered activity for hydrogenolysis and for ‘carbon’ formation have been examined.175−177 Using single-crystal surfaces of platinum modified by deposition of tin so as to give p(2 × 2) Sn/Pt(111) (i.e. Pt3Sn) and (√3 × √3)R30◦Sn/Pt(111) (i.e. Pt2Sn) (see Sections 3.2 and 12.2.2), it was found176 that rates of n-butane hydrogenolysis at all temperatures were Pt2Sn < Pt < Pt3Sn. Product selectivities were changed by tin to give much higher values of S2 and lower values of both S1 and S3, suggesting that the preferred route became that involving more dehydrogenated species (Scheme 13.3), but the results were only shown graphically, and as is usual for UHV systems were somewhat scattered. The activity of the corresponding Ni3Sn surface was also less than that of Ni(111) but the extent of deactivation by carbon was much reduced.177
The inclusion of indium with Pt/Al2O3 had similar effects to those of tin.175 Heats of adsorption of hydrogen were lowered and rates of n-butane hydrogenolysis were smaller by about 102, due mainly to a decrease in the pre-exponential factor. Isomerisation selectivities were somewhat increased, possible at the expense of S2; the weaker hydrogen adsorption encouraged routes for species more fully dehydrogenated (Scheme 13.3). Addition of indium to Pt/NaY zeolite on the hand increased hydrogen adsorption strength at 625 K, as the order in hydrogen became progressively more negative and that of n-butane more positive.178 Both indium and tin therefore act to lower the mean size of the reactive ensemble and to lessen excessive dehydrogenation, without any significant electronic effect.
13.8.4. Platinum and Iridium plus Zirconium, Molybdenum, and Rhenium
The advent of the bimetallic PtRe/Al2O3 catalyst revolutionised the practice of petroleum reforming, by reason chiefly of its greater stability and longevity. There have been several studies directed towards understanding what difference in the roles of the metallic function might contribute to the benefits in industrial practice (see Further Reading section). In the hydrogenolysis of ethane on Pt(111) modified by deposition of rhenium, orders in hydrogen became less negative at 623 K, signalling a weakening of hydrogen chemisorption,179 but interpretation of the kinetics obtained with Pt/Al2O3 and PtRe/Al2O3 led to the opposite conclusion.65 There were significant changes in product selectivities3,43,65 (Table 13.16). With propane, S2 decreased when rhenium was present, to an extent that depended on

580 CHAPTER 13
TABLE 13.16. |
Hydrogenolysis of Propane (T2 = S2 ) and of n-Butane |
(Kinetic and |
|||||||
|
3 |
||||||||
|
Selectivity Parameters) on Pt/A12 O3 and PtRe/A12 O3 Catalysts at 603 K |
|
|||||||
%Pt |
%Re |
T3 |
r 1 |
E2 |
S2 |
S3 |
F |
T3 |
Note |
0.3 |
0 |
0.992 |
0.4 |
134 |
0.32 |
0.83 |
0.16 |
0.99 |
a |
0.6 |
0 |
0.986 |
8.4 |
150 |
0.34 |
0.82 |
0.17 |
0.98 |
b |
0.3 |
0.3 |
0.844 |
5.9 |
145 |
0.79 |
0.45 |
0.39 |
0.74 |
c |
0.45 |
0.45 |
0.834 |
3.4 |
140 |
0.68 |
0.63 |
0.44 |
>1 |
d |
|
|
|
|
|
|
|
|
|
|
1 In mmol gcat−1 h−1 . 2 In kJ mol−1 .
Catalysts have been designated as follows: a CK303 (EUROPT-3); b CK306; c CK433 (EUROPT-4); d CK455.
the conditions of pre-treatment, which presumably affected surface composition; rates increased as S2 fell.3 With n-butane the methane selectivity increased with the rhenium/platinum ratio.180,181 There were observed numerous subtle differences with this reaction when Pt/Al2O3 and PtRe/Al2O3 were compared3,43,65 (Table 13.6), but two effects stood out: (i) the effect of thermal cycling on selectivities was much smaller with PtRe/Al2O3, showing that rhenium minimised ‘carbon’ deposition; and (ii) the splitting factor F was raised, and T3 lowered, when rhenium was present. These effects can all be attributed to an increase in the C M bond strength, for which the interpretation of rate dependences on hydrogen pressure by the ES5B equation provides some support.65
The relative values of the surface energies of platinum and of rhenium would suggest the former should segregate to the surface, but the particles are likely to be so small (1 to 1.5 nm) that there is effectively little or no interior, so the surface must contain atoms of both kinds, with the platinum atoms preferentially occupying low coordination-number sites.3,182 Now rhenium is a catalyst that is vigorous but non-selective for hydrogenolysis,6,149,180,181 but Pt1Re1/Al2O3 showed neither of these characteristics, appearing rather to look like a modified platinum catalyst. This view is supported by the common compensation line that the two types of catalyst share3,65 (Figure 13.23). Any surface rhenium atoms must therefore be so well dispersed that they cannot form ensembles large enough to display their true nature (Figure 13.24). Studies of PtRe/Al2O3 using IR spectroscopy of chemisorbed carbon monoxide183,184 and XANES184 suggest however that it is the rhenium atoms that segregate preferentially to the surface, probably occupying low CN sites. It is unclear why considerations of surface energy182 do not apply in this case; perhaps they are irrelevant for single atoms, and the character of bonding to adjacent atoms of the same or different kinds may be more important. XANES results184 do however confirm some mutual electronic influence.
Somewhat different conclusions have been reached in a study180,185 of n- butane hydrogenolysis on a series of PtRe/α-Al2O3 catalyst covering the whole composition range. There was an astronomic factor (× 103) between the rates shown by platinum and the Pt25Re75 catalyst (Figure 13.25), and as little as 12.5% rhenium was sufficient to increase methane selectivity and to move the Arrhenius

REACTIONS OF THE LOWER ALKANES WITH HYDROGEN |
581 |
Figure 13.23. Compensation plot of Arrhenius parameters for hydrogenolysis of propane (O) and of n-butane ( ) on Pt/Al2 O3 (EUROPT-3) and PtRe/Al2 O3 (EUROPT-4). The two sets of results (ref. 43, filled points; ref. 3, open points) are from independent studies on different batches of catalyst, published respectively in 1989 and 1996.
parameters away from the characteristic platinum line (Figure 13.26). Conversion to rhenium-type behaviour was complete at 37.5% rhenium. This difference may have been a consequence of the use of various forms of pre-treatment (see also Section 14.5.3). Under petroleum reforming conditions, PtRe/Al2O3 catalysts are sulfided, and the ReS species thus formed act only as inert diluents.
Remarkably large promotional effects have been observed when molybdenum was added to Pt/SiO1502 ,151,186,187 or Pt/NaY zeolite.188 The rate of ethane
hydrogenolysis was raised by 102 and activation energy lowered from 226 to 138 kJ mol−1; the order in hydrogen became less negative, suggesting a weakening of its chemisorption.150 The opposite effect has however been found with n-butane,151,187 where a similar increase in rate achieved its maximum at 40%
Figure 13.24. Models of metal particles containing 46 platinum atoms (size 1.2 nm, 72% dispersion) or 23 atoms each of platinum and rhenium: of the 13 interior atoms, all are rhenium, and of the remaining 10 there are six visible (shaded).3

582 |
CHAPTER 13 |
Figure 13.25. Compensation plot for n-butane hydrogenolysis on PtRe/α-Al2 O3 catalysts of various compositions:180,185 filled point, Pt; open points, 50–100% Re; half-filled points, intermediate values.
molybdenum. Examination of Arrhenius parameters suggests that PtMo/SiO2 catalysts containing 12 to 50% molybdenum belonged to a separate more active class, while at higher concentrations there was another less active class. Rates expressed per hydrogen atom adsorbed did not however decrease.151 The cause of the effect has not been established for certain, but it seems possible that Mo5+ ions decorate the platinum surface, and that a ‘bimetallic’ site is more active than a purely platinum site. Skeletal isomerisation was suppressed and S2 increased. Analogous effects have also been seen187 with PdMo/SiO2 and with PtW/SiO2. IrMo/Al2O3
Figure 13.26. Activity of PtRe/α-Al2 O3 catalysts for n-butane hydrogenolysis as a function of rhenium content at 513 K (ln (rate/molecules cm−2 s−1 )).180,185

REACTIONS OF THE LOWER ALKANES WITH HYDROGEN |
583 |
(Ir3Mo) prepared from the bimetallic carbonyl complex was somewhat more active for the n-butane reaction at 488 K than a pure iridium catalyst,189 but high ethane selectivity was retained (1.43). The Ir2Mo2 complex gave a less active catalyst, with a lower value of S2 (1.08). The addition of zirconium to platinum (Pt75Zr25/C) lowered the rate of ethane hydrogenolysis about 20-fold, and raised the activation energy.152
13.8.5. Bimetallic Catalysts of Metals of Groups 8 to 10
As we have noticed previously, results of work done with bimetallic catalysts having components drawn from within Groups 8 to 10 rarely cause much excitement; they are usually intermediate between those shown by each metal separately (although synergism of rates is quite commonly met48), and what has been done with alkane hydrogenolysis is no exception. There is little evidence for bimetallic sites giving effects radically different from those shown by monometallic sites. Systems examined include iron-platinum,40,190 rhodium-platinum191,192 and iridium-platinum,48,163,193 but studies of the latter have done little to illuminate its particular virtues for petroleum reforming. The rhodium-iridium194–195 and ruthenium-iridium171 systems have also been looked at, using n-butane as the reactant: in the nickel-rhodium system,10,197 the high ethane selectivity characteristic of rhodium grows with the rhodium content, reaching a value of 1.4 at Ni60Rh40. Other combinations (e.g. Ir-Os185) have shown marked synergism.
13.9. APOLOGIA
The extensive attention that has been paid to the reactions of the lower alkanes on metal catalysts reflects the wide range of phenomena encountered with structure-sensitive reactions, as opposed to those reactions met with earlier, the insensitivity of which limited the importance of variables such as particle size, crystal face and composition of bimetallic systems. Far more attention has also been paid to the careful measurement of reaction kinetics, and their interpretation by various models. This, one hopes, explains even if it does not excuse the length of this chapter.
REFERENCES
1.G.C. Bond, Appl. Catal. A: Gen. 148 (1997) 3.
2.Z. Pa´al and P. T´et´enyi in: Specialist Periodical Reports: Catalysis, (G.C. Bond and G. Webb, eds.), Roy. Soc. Chem. Vol.5 (1982), p. 80.
3.G.C. Bond and R.H. Cunningham, J. Catal. 163 (1996) 328.

584 |
CHAPTER 13 |
4.G.C. Bond and Xu Lin, J. Catal. 168 (1997) 207; 169 (1997) 76.
5.G.C. Bond and Xu Lin, J. Catal. 169 (1997) 76.
6.G.C. Bond and M.R. Gelsthorpe, Catal. Lett. 2 (1989) 257; J. Chem. Soc. Faraday Trans. I 87 (1991) 2479.
7.S.L. Anderson, J. Szanyi, M.T. Paffett and A.K. Datye, J. Catal. 159 (1996) 23.
8.J.R. Engstrom, D.W. Goodman and W.H. Weinberg, J. Am. Chem. Soc. 110 (1988) 8305; 108 (1986) 4653.
9.K. Foger and J.R. Anderson, J. Catal. 59 (1979) 325.
10.G. Leclercq, S. Pietrzyk, J.F. Lamonier, L. Leclerq, L.M. Bouleau and R. Maurel, Appl. Catal. A: Gen. 123 (1995) 161.
11.Z. Pa´al and P. T´et´enyi, Nature 267 (1977) 234.
12.G. Leclercq, L. Leclercq and R. Maurel, J. Catal. 44 (1976) 68; 50 (1977) 87.
13.G.C. Bond, J. Catal. 115 (1989) 286.
14.G.C. Bond and Lou Hui, J. Catal. 137 (1992) 462.
15.S. Gao and L.D. Schmidt, J. Catal. 111 (1988) 210; 115 (1989) 356.
16.L. Guczi, K. Matusek, A. S´ark´any and P. T´et´enyi, Bull. Soc. Chim. Belg. 88 (1979) 497.
17.S.D. Jackson, G.J. Kelly and G. Webb, J. Catal. 176 (1998) 225.
18.Z. Pa´al, Adv. Catal. 29 (1980) 273.
19.L. Bonneviot and G.L. Haller, J. Catal. 130 (1991) 359.
20.G. Leclercq, L. Leclercq, L.M. Bouleau, S. Pietrzyk and R. Maurel, J. Catal. 88 (1984) 8.
21.M.F. Guilleux, J.A. Dalmon and G.A. Martin, J. Catal. 62 (1980) 235.
22.E.I. Ko and P.A. Burke, J. Catal. 127 (1991) 453.
23.J.H. Sinfelt, Catal. Today, 53 (1999) 303.
24.J.H. Sinfelt, Adv. Catal. 23 (1973) 91.
25.J.H. Sinfelt Catal. Rev. 3 (1969) 175.
26.J.H. Sinfelt and W.F. Taylor, Trans. Faraday Soc. 64 (1968) 3086.
27.J.H. Sinfelt, W.F. Taylor and D.J.C. Yates, J. Phys. Chem. 69 (1965) 95.
28.J.H. Sinfelt and D.J.C. Yates, J. Catal. 8 (1967) 82.
29.J.H. Sinfelt and D.J.C. Yates, J. Catal. 10 (1968) 362.
30.G.C. Bond, Catal. Today 49 (1999) 41.
31.P. T´et´enyi, L. Guczi and A. S´ark´any, Acta Chim. Acad. Sci. Hung. 97 (1978) 221.
32.L. Guczi, A. S´ark´any and P. T´et´enyi J. Chem. Soc. Faraday Trans. I 70 (1974) 1971.
33.G.C. Bond, Appl. Catal. A: Gen. 191 (2000) 23.
34.F.H. Ribeiro, A.E. Schach von Wittenau, C.H. Bartholomew and G.A. Somorjai, Catal. Rev.-Sci. Eng. 39 (1997) 49.
35.G.A. Somorjai, Introduction to Surface Chemistry and Catalysis, Wiley: New York (1994).
36.P.A. Burke and E.I. Ko, J. Catal. 116 (1989) 230.
37.C.J. Machiels and R.B. Anderson, J. Catal. 58 (1979) 253, 260, 268; 60 (1979) 339.
38.A. S´ark´any, K. Matusek and P. T´et´enyi, J. Chem. Soc. Faraday Trans. I 73 (1977) 1699.
39.J.R. Anderson and N.R. Avery, J. Catal. 5 (1966) 446.
40.B.S. Gudkov, L. Guczi and P. T´et´enyi, J. Catal. 74 (1982) 207.
41.G.C. Bond and Xu Yide, J. Chem. Soc. Faraday Trans. I 80 (1984) 969.
42.J.C. Kempling and R.B. Anderson, Proc. 5t h Internat. Congr. Catal., (J.W. Hightower, ed.), North Holland: Amsterdam 2 (1972) 1099.
43.G.C. Bond and M.R. Gelsthorpe, J. Chem. Soc. Faraday Trans. I, 85 (1989) 3767; Catal. Lett. 3 (1989) 359.
44.D.F. Hollis and H. Taheri, AIChE Journal 22 (1976) 1112.
45.J.J. Burton and E. Hyman, J. Catal. 37 (1975) 114; J.J.Burton, E. Hyman and B.G. Fedak, J. Catal. 37 (1975) 106.
46.R.D. Cortright, R.M. Watwe, B.E. Spiewak and J.A. Dumesic, Catal. Today 53 (1999) 395.