
Metal-Catalysed Reactions of Hydrocarbons / 08-Hydrogenation of Alkenes and Related Processes
.pdf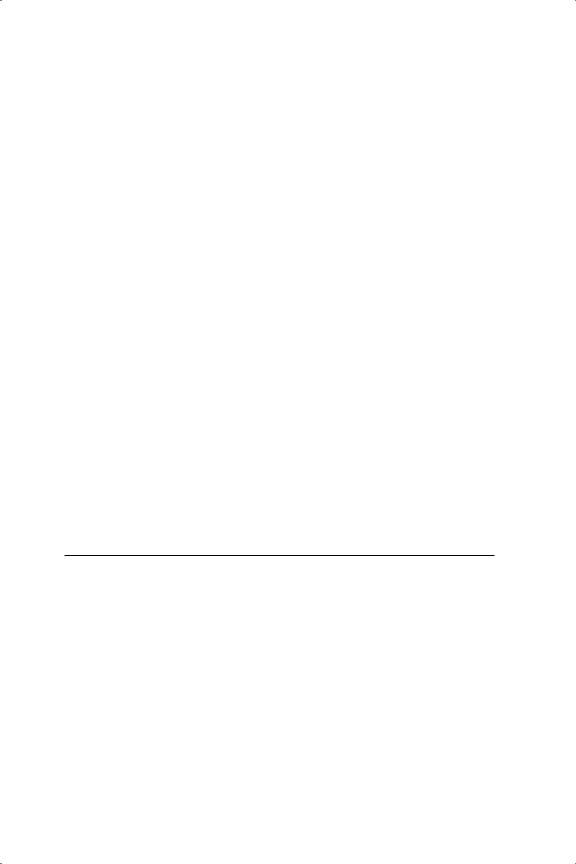
HYDROGENATION OF ALKADIENES AND POLY-ENES |
367 |
170 K,45 butadiene was adsorbed in a di-σ form, which may have been 1,2-di-σ as suggested by VEELS, or 1,4-di-σ (i.e. CH2––CHCH––CH2 ) as indicated by NEXAFS:46 this latter technique identifies the structure as being parallel to the surface, which the 1,2-di-σ form would not be. The methods agreed that a more strongly held form occurred at 300 K, and this may have been the 1,2,3,4-tetra-σ state. On Pd(111) however NEXAFS measurements46 also showed a form in which the plane of the species was parallel to the surface, but it was clearly a di-π structure, which is in keeping with what is known about alkenes (Chapter 7): it also changed to a more firmly attached but unidentified form at 300 K. The low temperature structures may well have some relevance to those involved in hydrogenation above about 273 K, but the intermediate species may not be bonded to the surface in the same way as the reactant.
8.3.3. Hydrogenation of 1,3-Butadiene on Single Crystal Surfaces
There have been correspondingly few papers describing this reaction on single crystal surfaces of metals.47−50 Four concern the reaction on various surfaces of platinum, but their value is unfortunately somewhat limited; two of them49,50 used both Pt(100) and Pt(111), but TOFs were given at quite different temperatures; with another pair47,48 it is only possible to compare results on Pt(110). While activation energies (43 ± 10 kJ mol−1) and order of reaction where determined were broadly comparable (Table 8.4), values of TOF sometimes showed a marked variation between different surfaces, and it is not possible to define a unique hierarchy of activity. Most disappointingly there is little information on product selectivities apart from Stot (Table 8.4): in one publication49 only partial information was
TABLE 8.4. Kinetic Parameters for the Hydrogenation of 1,3-Butadiene on Various Single Crystal Faces of Platinum, and on Platinum Foil
Face/Form |
T /K |
H2 /C2 H4 |
TOF/s−1 |
x |
y |
E /kJ mol−1 |
Stot |
References |
(100) |
300 |
10 |
0.27 |
— |
— |
— |
— |
49 |
|
298 |
— |
2 |
— |
— |
42 |
54 |
47 |
|
373 |
25 |
11 |
0.5 |
0 |
33,53 |
52 |
50 |
(110) |
298 |
— |
4 |
— |
— |
42 |
64 |
47 |
|
373 |
25 |
28 |
2 → 1 |
0 |
39 |
— |
50 |
(111) |
300 |
10 |
85 |
— |
— |
— |
— |
49 |
|
373 |
25 |
8 |
1 |
1 → 0 |
38,39 |
40,67 |
50 |
(755) |
300 |
10 |
0.71 |
1.12 |
−0.13 |
41 |
62 |
49 |
Foil |
300 |
10 |
0.9 |
1.16 |
−0.10 |
41 |
60 |
49 |
Note: (i) Differences in TOF at 300 K may be partly due to use of different pressures of reactants.
(ii) Temperatures are those at which TOFs were measured; the orders may have been found at slightly different values.
Orders change as shown as pressure of variable increases.

368 |
CHAPTER 8 |
provided, and in the others there were major gaps or only a qualitative statement. Failure to record the most pertinent and important results is to be deeply regretted.
When hydrogen + deuterium mixtures were used in the reaction,48,50 the rate of isotope equilibration was more than 10 times faster than butadiene hydrogenation, and had an activation energy of 17 kJ mol−1.48 Apart from showing that equilibration can proceed in parallel with hydrogenation, this mode of experimentation has little to commend it. Stot decreased with increasing ‘hydrogen’ pressure below 125 Torr, but then levelled out: neither it nor the activation energy was affected by adsorbed carbon formed by deep dehydrogenation of adsorbed hydrocarbon species, and the rate depended only on the area of clean surface.48 On Pt(100), coverages by potassium and sodium up to 40% had only slight positive effects on rate and Stot.47 Poisoning of the (110) surface by sulfur caused the rate to decrease linearly with coverage, one sulfur atom blocking one site for hydrogen dissociation.51 Activation energy and mechanism were unchanged, and there was no indication of an effect on product selectivities.
On Pd(100)(1 × 1) precoated with either hydrogen or deuterium atoms, temperature-programming showed that butadiene was hydrogenated with 100% selectivity to butenes.36 The Pd(110) face was almost ten times more active than the (111) face at 300 K;15,52 species intermediate in the hydrogenation have been examined on the (110) surface.53
Work with bimetallic single crystal surfaces will be mentioned in Section 8.3.7.
8.3.4.Hydrogenation of 1, 3-Butadiene on Supported and Unsupported Metals
This reaction has been examined in great depth on a number of supported and unsupported metals in various laboratories,19,14,54−58 and extensive use of deuterium for isotopic labelling has led to very detailed mechanistic schemes.10,38,59 In this section we focus on the products formed with hydrogen, and the effect of catalyst composition and structure thereon: studies bearing directly on detailed mechanism are deferred to the next section.
As noted in Section 8.3.1, measurements of orders of reaction and activation energies indicate strong adsorption of butadiene to the exclusion of butenes, and little if any competitive adsorption of hydrogen; activation energy values are usually low, as befits true values, the surface concentrations being invariant with temperature. Any departure from these norms is therefore noteworthy and Table 8.5 contains a selection of results for alumina-supported metals, some of which are ‘usual’ and a few that are not. Of the latter kind, we may note that hydrogen order x may be significantly greater than unity, while simultaneously the orders in butadiene y are somewhat negative (Co, Pd, Pt). Somewhat higher activation energies have sometimes been reported (Pd, Pt), while a low value was associated
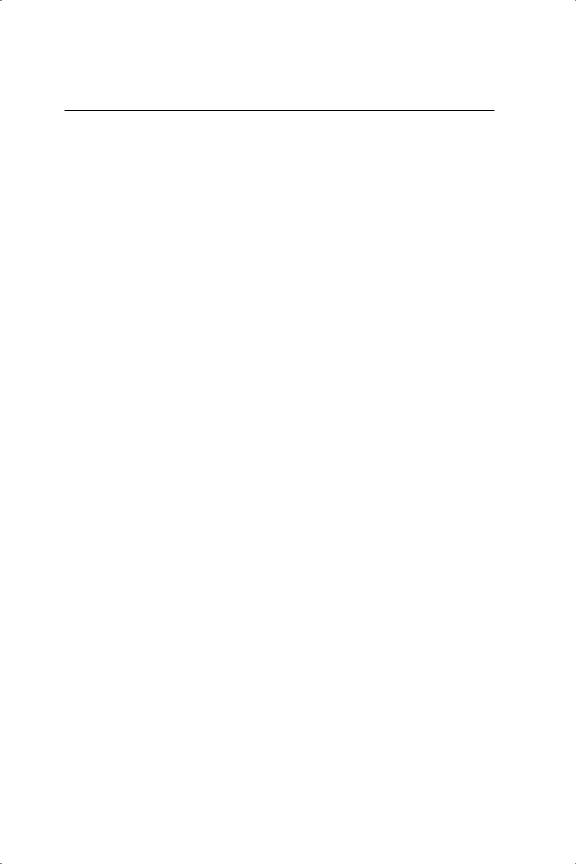
HYDROGENATION OF ALKADIENES AND POLY-ENES |
369 |
TABLE 8.5. Kinetic Parameters for the Hydrogenation of 1,3-Butadiene
Metal |
Form |
x |
y |
T /K |
E /kJ mol−1 |
References |
Fe |
/A12 O3 |
— |
— |
— |
46 |
59 |
Co |
/A12 O3 |
1.0 |
0 |
433 |
36 |
59 |
Co |
Powder |
1.5 |
−0.5 |
441 |
51 |
59 |
Ni |
Powder |
1.3 |
0.8 |
473 |
52 |
66 |
Ni |
/A12 O3 |
1.0 |
−0.2 |
351 |
63 |
59 |
Ru |
/A12 O3 |
1.0 |
0 |
287 |
52 |
65 |
Rh |
Sponge |
1.0 |
— |
383 |
31 |
96 |
Rh |
/A12 O3 |
1.0 |
0.1 |
273 |
46 |
65 |
Pd |
/A12 O3 |
1.7 |
−0.7 |
289 |
70 |
65 |
Pd |
/A12 O3 |
1 |
0 |
295 |
85 |
129 |
Pd |
/Pumice |
0.9 |
0 |
293 |
44 |
63 |
Pd |
Sponge |
1.2 |
— |
383 |
48 |
96 |
Ir |
/A12 O3 |
0.8 |
0 |
273 |
19 |
65 |
Pt |
/A12 O3 |
1.3 |
−0.5 |
289 |
81 |
65,75 |
Cu |
/A12 O3 |
1.0 |
0 |
378 |
55 |
65 |
Ag |
/SiO2 |
— |
— |
345 |
38 |
60 |
Au |
/A12 O3 |
1.0 |
0 |
473 |
36 |
130 |
with a low Stot in the case of iridium. The base metals (Fe, Co, Ni, Cu) together with palladium, silver60 and gold54,61,62 showed values of Stot that were very close if not exactly equal to unity (Table 8.6). Such values were generally not temperature dependent,63 nor did they vary much with hydrogen pressure. With the other metals, high values of Stot were approached as hydrogen pressure was lowered or temperature raised. Both these trends would have given lower concentrations of hydrogen atoms on the surface; we must therefore deduce that (notwithstanding the values of the orders) some hydrogen is adsorbed alongside the butadiene on the noble metals of Groups 8 to 10 (except palladium, which always appears exceptional).
Values of kinetic parameters and Stot are also available for films,54 wires64 and powders:59,64 the principal difference in the case of wires lies in the necessarily much higher temperatures that had to be used. With rhodium, iridium and platinum, this had the consequence that very much greater values of Stot were found, while orders and activation energies were generally similar to those in Table 8.6. Reasons for these high selectivities will be considered later. In the case of metal films, where values for Stot are available for the majority of metals in Groups 3 to 11, mostly at 273–293 K; nearly all exceed 95%, exceptions being the metals of Group 6 (Cr, 90%; Mo, 84%; W, 90%), rhenium (80%) and most notably platinum (59%).
We come now to the matter of the relative amounts of the butene isomers formed, expressed as S1, SZ 2 and SE 2. Except for the base metals of Groups 8 to 10, where special factors apply, it is fairly easy to quote typical values for each metal, because in general they were only weakly dependent on temperature (unless
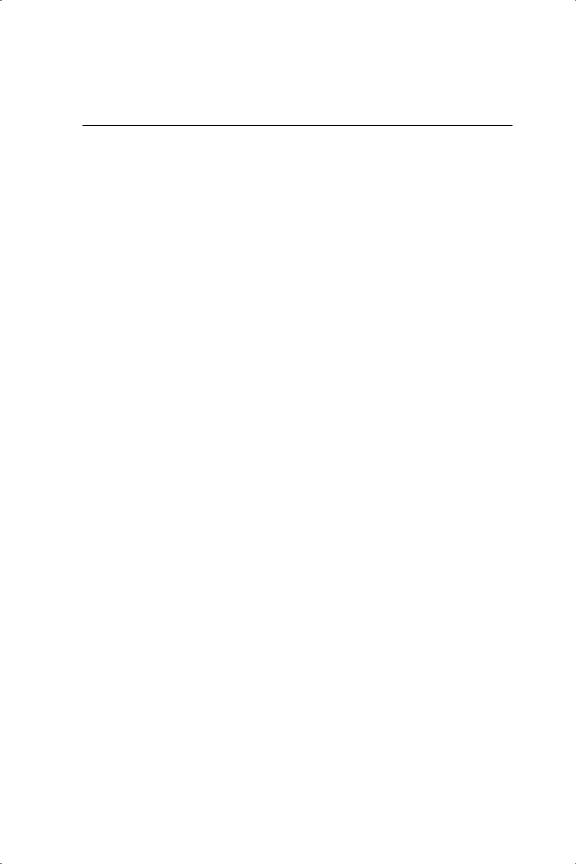
370 CHAPTER 8
TABLE 8.6. Hydrogenation of 1,3-Butadiene: Product Selectivities for Various Forms of Metal Catalysts
Metal |
Form |
Stot |
S1 |
SZ 2 |
SE 2 |
Type |
T /K |
References |
Fe |
/A12 O3 |
0.98 |
23 |
32 |
45 |
B |
471 |
59 |
|
Film |
0.97 |
69 |
12 |
19 |
A |
273 |
54 |
Co |
/A12 O3 |
1.00 |
28 |
8 |
64 |
B |
348 |
59,63 |
|
/A12 O3 |
— |
74 |
17 |
9 |
A |
650 |
100 |
|
/A12 O3 |
— |
82 |
7 |
11 |
A |
333 |
14 |
|
/A12 O3 |
0.98 |
72 |
10 |
18 |
A |
573 |
131,132 |
|
Powder |
1 |
72 |
10 |
18 |
A |
573 |
59 |
|
Film |
1 |
70 |
10 |
20 |
A |
297 |
54,66 |
Ni |
/A12 O3 |
1.00 |
26 |
8 |
66 |
B |
373 |
40,59 |
|
/A12 O3 |
0.95 |
64 |
11 |
25 |
A |
296 |
133 |
|
/SiO2 |
— |
59 |
12 |
29 |
A |
373 |
40 |
|
/SiO2 |
0.96 |
61 |
14 |
25 |
A |
353 |
133 |
|
Powder |
— |
62 |
13 |
25 |
A |
373 |
14,40,59,66 |
|
Film |
1 |
69 |
9 |
22 |
A |
273 |
54 |
Ru |
/A12 O3 |
0.74 |
69 |
12 |
19 |
— |
273 |
14 |
|
/A12 O3 |
0.90 |
57 |
22 |
21 |
— |
293 |
56 |
Rh |
/A12 O3 |
0.74 |
51 |
17 |
32 |
— |
289 |
65 |
|
/A12 O3 |
0.89 |
51 |
21 |
28 |
— |
293 |
56 |
|
Film |
0.92 |
59 |
10 |
31 |
— |
265 |
65 |
|
Wire |
0.99 |
38 |
25 |
37 |
— |
488 |
64 |
Pd |
/BaSO4 |
0.94 |
49 |
11 |
40 |
— |
261 |
44 |
|
/A12 O3 |
1.00 |
65 |
2 |
33 |
— |
273 |
14,65 |
|
/A12 O3 |
1.00 |
67 |
5 |
28 |
— |
273 |
8 |
|
/A12 O3 |
1.00 |
53 |
5 |
42 |
— |
308 |
25 |
|
/A12 O3 |
1.00 |
60 |
5 |
35 |
— |
298 |
132 |
|
/A12 O3 |
0.98 |
58 |
10 |
32 |
— |
293 |
56 |
|
/Pumice |
1.00 |
48 |
4 |
48 |
— |
292 |
63 |
|
/Pumice |
0.99 |
78 |
3 |
19 |
— |
273 |
93 |
|
Film |
0.98 |
61 |
6 |
33 |
— |
198 |
54 |
|
Wire |
1.00 |
41 |
16 |
43 |
— |
408 |
64 |
|
Sponge |
1 |
56 |
10 |
34 |
— |
458 |
94 |
|
h.f. |
0.995 |
99 |
0.5 |
0.5 |
— |
313 |
134 |
Os |
/A12 O3 |
0.43 |
65 |
16 |
19 |
— |
297 |
135 |
Ir |
/A12 O3 |
0.25 |
59 |
22 |
19 |
— |
297 |
135 |
|
Wire |
0.92 |
58 |
22 |
20 |
— |
465 |
54 |
Pt |
/A12 O3 |
0.50 |
72 |
10 |
18 |
— |
273 |
135 |
|
/A12 O3 |
0.60 |
65 |
13 |
22 |
— |
273 |
75,136 |
|
/A12 O3 |
0.78 |
82 |
6 |
12 |
— |
298 |
76 |
|
/A12 O3 |
0.53 |
68 |
21 |
23 |
— |
293 |
56 |
|
/SiO2 |
0.68 |
78 |
8 |
14 |
— |
290 |
75,136 |
|
Film |
0.59 |
72 |
9 |
19 |
— |
273 |
54 |
|
Wire |
0.99 |
46 |
28 |
26 |
— |
538 |
64 |
|
Powder |
— |
77 |
10 |
14 |
— |
273 |
97 |
|
(111) |
— |
77 |
8 |
15 |
— |
393 |
50 |
|
Foil |
— |
67 |
13 |
20 |
— |
300 |
49 |
|
|
|
|
|
|
|
|
(continued ) |
|
|
|
|
|
|
|
|
|
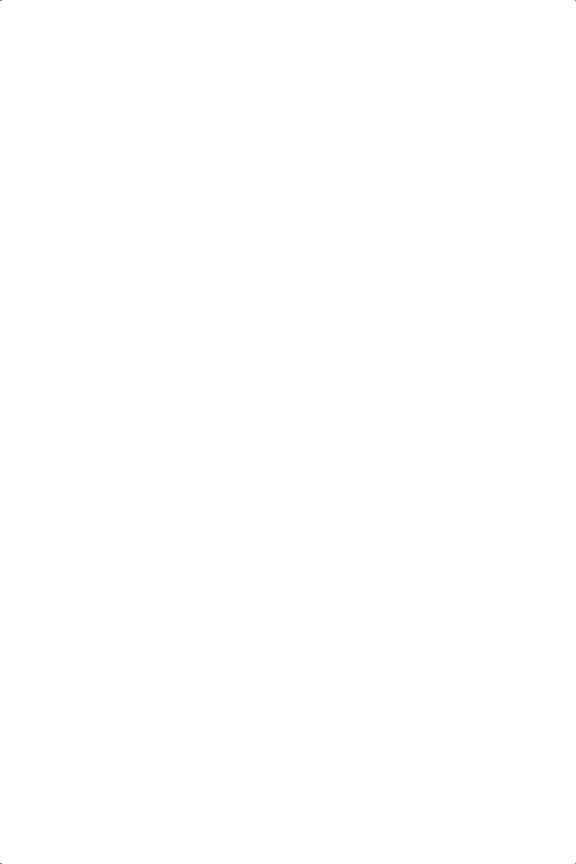
HYDROGENATION OF ALKADIENES AND POLY-ENES |
371 |
|
|
|
TABLE 8.6. |
(Continued) |
|
|
|
|
Metal |
Form |
Stot |
S1 |
SZ 2 |
SE 2 |
Type |
T /K |
References |
Cu |
/A12 O3 |
1.00 |
87 |
7 |
6 |
— |
333 |
59,135 |
|
/SiO2 |
1.00 |
82 |
9 |
9 |
— |
295 |
129 |
|
Film |
0.99 |
78 |
14 |
8 |
— |
383 |
54 |
Ag |
Film |
0.96 |
50 |
20 |
30 |
— |
415 |
54 |
|
/SiO2 |
1.00 |
66 |
10 |
24 |
— |
345 |
60 |
Au |
/A12 O3 |
1.00 |
59 |
28 |
13 |
— |
443 |
10,135 |
|
Film |
1.00 |
73 |
11 |
16 |
— |
431 |
54 |
|
|
|
|
|
|
|
|
|
Note: The above results have been obtained at various H2 /butadiene ratios and degrees of conversion, but neither has a major effect.
Metal inside hollow fibre.
unusually high, as for wires), and on conversion or hydrogen pressure,54 save for those cases where Stot was significantly below unity. There the isomers interconvert and move towards their equilibrium proportions at rates that parallel the change in the hydrogen/butadiene ratio that occurs with increasing conversion if their ratio is initially more than unity.65 This occurs a fortiori the higher the temperature, and confirms the presence of some competitively adsorbed hydrogen. Product selectivities observed at conversions where these complications are absent are shown in Table 8.5; some values from different laboratories are included to emphasise the universality of what is found, but the cited publications contain many more values for which we have no space. Butene selectivities for the metal films are plotted against Periodic Group number in Figure 8.5.
Although it may seem at first sight that the values of the selectivities are almost random, closer inspection reveals some important regularities. (1) The Z - 2-butene selectivity is almost always the smallest; in the case of palladium at about room temperature (under which conditions the β-hydride phase may be formed) it is 5% or less. (2) 1-Butene is almost always the major product, and with copper, manganese and cobalt (Type A) it is more than 85%. Only with zirconium, and with iron, cobalt and nickel as Type B, is E-2-butene formed as major product.
(3) Except for the base metals of Groups 8 to 10, product distributions under comparable conditions show little significant dependence on physical form, as befits a structure-insensitive reaction.
With these base metals, the dependence of the form of product distribution on the kind of support used (if any) (Table 8.6), and on reduction temperature, was for a long time a major puzzle:26,40,63 it was finally decided that the trouble with alumina was due to sulfate ions in the support being reduced by hydrogen spillover to hydrogen sulfide, which partially covered the metal with sulfur atoms, causing Type B behaviour.14,66 Similar effects had been seen with 1,2-butadiene.26 The mechanism by which this happened is mentioned in the next section. This idea has been comprehensively confirmed by depositing sulfur atoms onto metal films although the obvious ploy of using alumina made by a sulfate-free route has

372 |
CHAPTER 8 |
Figure 8.5. Product selectivities in the hydrogenation of 1,3-butadiene on metal films (data for Ir are for wire):54 1-butene, open bar; E -2–butene, hatched bar; Z -2-butene, filled bar.
not been tried. With manganese and nickel films, there was a progressive trend from Type A to Type B distributions as sulfur coverage was increased, and fully sulfided films of a number of Groups 6 to 10 metals all (except Pt) showed Type B behaviour, and very high values of Stot. On platinum it appeared to act as a nonselective poison;48,51,54 iron and cobalt films showed typical Type A behaviour. Other electronegative additives (P, As, Se) applied to Co/Al2O3, and halogens applied to nickel powder had similar effects.14,66 Hydrided films of metals in Groups 3 to 5 also mainly showed Type B characteristics,14,54 because hydrogen atoms tend to withdraw charge from the metal atoms.
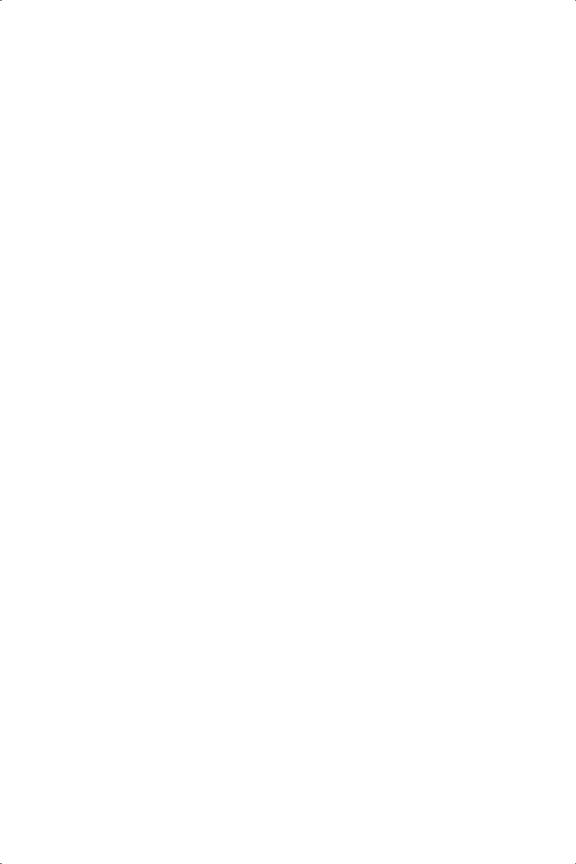
HYDROGENATION OF ALKADIENES AND POLY-ENES |
373 |
The high selectivity that is characteristic of palladium and certain other metals (Table 8.6) appears to be a consequence of the rapid formation of strongly adsorbed oligomers (C8, C12 etc) that probably act by decreasing the mean size of the active ensembles.15,67−69 Maintenance of high selectivity therefore depends upon keeping to conditions where they are retained, and using too high a hydrogen pressure is therefore not helpful. Too much oligomer formation is however also inimical to high butene selectivity, because three-dimensional carbonaceous islands are formed, and these restrict the movement of reactant and product to and from the active centres, leading to loss of selectivity. Butene isomer ratios were also changed,68 and activation energies decreased to 20–25 kJ mol−1 under these circumstances. Non-stoichiometric PdCx can also be formed with palladium black;68 this is less likely to occur with small particles, as is hydride formation. The role47,70,71 of added alkali metals has also been explored.
To prepare the ground for discussion of mechanisms, some further regularities may be noted: these are most clearly seen in the results for films (Table 8.6 and Figure 8.5), where complications due to adventitious poisons, support and particle size effects are likely to be circumvented. Two trends in particular have been noted.
(1) Values of S1 tend to increase on passing from left to right across the Periodic Table, although they are generally larger for the 3d metals than for the 4d and 5d metals. For these last two series, values of S1 have been related specifically to the Pauling electronegativity. (2) There is a kind of inverse correlation between S1 and SE 2/SZ 2; at least this ratio is less than three for all ‘pure’ metals, while those that are affected by sulfur show higher values. The exception is again palladium, where room temperature values can be as high as 16 (Table 8.6). It therefore seems that, because SZ 2 is uniformly low, it is the ratio of S1/SZ 2 that is the principal variable; they are mechanistically linked, and one can substitute for the other.
As noted above, the total selectivity to butenes Stot increases with temperature for those metals where it is not already near unity,64 increasing values approaching unity when wires are used at high temperatures (Table 8.6). Now for alumina– supported metals of Groups 8 to 10, the value of Stot at 298 K in each group (Table 8.6) falls within increasing atomic number, viz.
Fe > Ru > Os; Co > Rh > Ir; Ni = Pd > Pt.
Serendipitous use of measurements of hydrogen retained by metal powders formed by hydrogen reduction of salts has provided a likely explanation for these trends: except for iron (no datum) and palladium (exothermic absorption), the trends were the opposite of those above. The amounts occluded are however greater by large factors than those due to simple dissolution, and the metals in question (unlike those in Groups 4 and 5) do not form salt-like hydrides. It was therefore
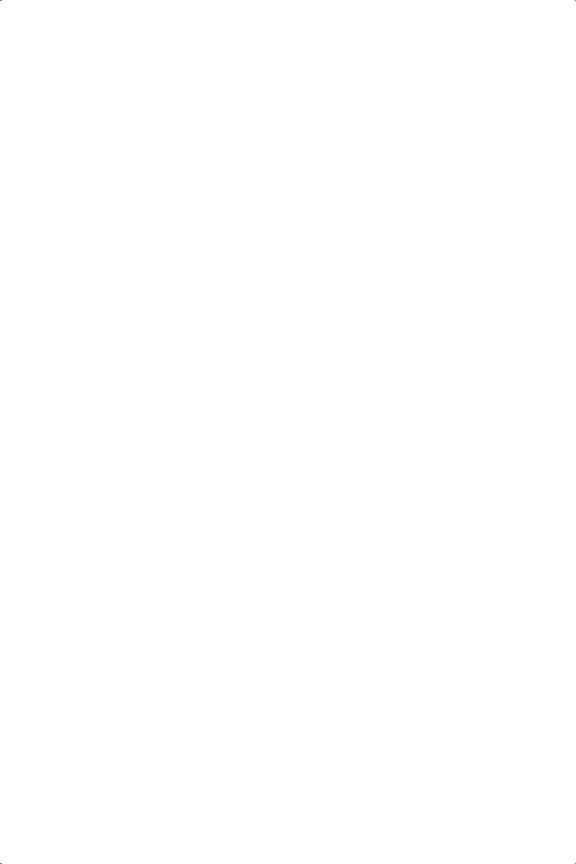
374 |
CHAPTER 8 |
supposed that the hydrogen is held in cavities that result from the failure of atoms formed in reduction of the salt to relax into the perfect metal lattice, the more so as their mobility at the reduction temperature TR decreases because their melting temperature TM rises. The amount of occluded hydrogen should therefore increase with TM or with Huttig temperature, which is one-third of TM and the point at which surface mobility becomes significant. This is qualitatively what is observed, and it was then believed that this extra hydrogen became activated by some means not fully explained, and contributed to the non-selective reduction of butadiene. This would also explain the effect of temperature on Stot, assuming that part of the occluded hydrogen is released as temperature is increased. This model received strong support from work on iridium powders,72,73 which gave Stot more than 95% even at 273 K when the H/Ir ratio was not more than 0.03. Furthermore the value of Stot rose as the dispersion of Ir/SiO2 and Ir/Al2O3 decreased; the extent of cavitation is expected to vary inversely with particle size. This is a subtle manifestation of a particle size effect that may apply in other systems; but platinum films and single crystals also show quite low values of Stot even at low temperatures.49,54
There have been several studies aimed at detecting support effects. With a series of supports of increasing acidity (MgO, Al2O3,SiO2-Al2O3), Stot, S1 and the E/Z ratio all decreased somewhat,74 but the effects were not large. It is of course possible that they were due to other causes such as a particle size effect or partial sulfur poisoning; it is easy but unwise to draw too hasty conclusions. Some unconventional supports have been used. Pt/MoO3 gave almost the same results as Pt/SiO2;75 Pt/Nb2O5 after reduction at 573 K or 773 K was less active than Pt/Al2O3, but product distributions were similar:76 there is thus no real evidence for a strong metal-support interaction affecting the reaction.77 With Pd/ZnO, reduction above 423 K led to lower rates but an increase in S1:78 some partial reduction of the support, followed by surface or bulk alloy formation, would occur under these conditions. To obtain high values of Stot it is necessary for there to be no constraint on the diffusion of the butenes away from the active sites; the location of the metal within a porous catalyst particle is therefore of critical importance.79,80
This point has been reinforced dramatically by work using palladium introduced into hollow fibres of cellulose acetate or polysulfone (Table 8.6); not only was Stot close to 100%, but the predominant product (96–99%) was 1-butene, with small and roughly equal amounts of the 2-butene isomers. It is hard to understand this result (which passed without comment or reference to the literature) in terms of diffusional effects; it seems more likely that the palladium was in a state such that only one of the two double bonds could be chemisorbed at a time. A palladium-cobalt catalyst made similarly behaved likewise, but was unable to isomerise 1-butene, which the palladium catalyst could.
Although in general product selectivities are much the same for small supported particles as for large unsupported ones, there is much evidence to show that small palladium particles are less active than large ones.70,81−84 This conclusion

HYDROGENATION OF ALKADIENES AND POLY-ENES |
375 |
applies both to particles made by atomic beam deposition or by plasma sputtering onto silica,81 silicon carbide82 or graphite83,85 and to those made chemically:84,86 particles less than 1 nm in size were found to be inactive, while those larger than about 2 nm had the same order of activity as single crystal surfaces.81,83 Those made by the atomic beam method appeared however to be more susceptible to deactivation by carbon deposition than those made chemically, and there have been indications that high Stot is a consequence of this, or even of carbide formation. The low activity of very small particles has been blamed on the excessively strong adsorption of butadiene on low coordination number atoms: remembering that such small particles may not show their metallic character (Section 2.5), we expect their atoms to behave more as individuals, and hence to be able to form complexes with alkadienes analogous to those they can form with single atoms and ions. The increase in the Pd 3d binding energy as size decreases is another symptom of this effect.52,70
8.3.5.The Reaction of 1, 3-Butadiene with Deuterium: Reaction Mechanisms
The material presented in the last section provides ample scope for a serious discussion of reaction mechanisms, but there is one remaining input that needs to be addressed: as always, the use of deuterium in place of hydrogen and identifying the locations of deuterium atoms in the products provides an additional constraint on the possibilities, and often suggests unsuspected pathways. The reaction of butadiene with deuterium has been studied on alumina-supported cobalt, nickel, copper, rhodium, palladium and platinum.25,38,59 Product analyses have been made at different conversions, but the exigencies of the work have precluded detailed investigation of variables such as temperature and reactant pressures.59 Each analysis required the separation of five hydrocarbons by preparative gas chromatography and the mass-spectroscopic analysis of each one; the tedium of this procedure readily excuses the absence of more extensive work. What has been reported is however quite enough to be helpful; perhaps when sanity returns to the funding of scientific research, some of the many remaining gaps will get filled.
The main conclusions are similar for all the metals studied. There was limited but variable exchange of the reactant’s hydrogen atoms (Table 8.7), and only small amounts of hydrogen appeared in the deuterium,38,59 showing that the concentration of atoms on the surface under the conditions of the experiments was small. The distributions of deuterium in each of the butenes were broadly the same, showing that each was an initial product, and not formed by the isomerisation of any other: thus both 1,2- and 1,4-addition processes operate. The mean deuterium number M for each butene isomer increased with conversion to an extent and in a manner that reflected how exchange in the butadiene exceeded that in the deuterium: a selection of initial values is shown in Table 8.7. Little purpose is served
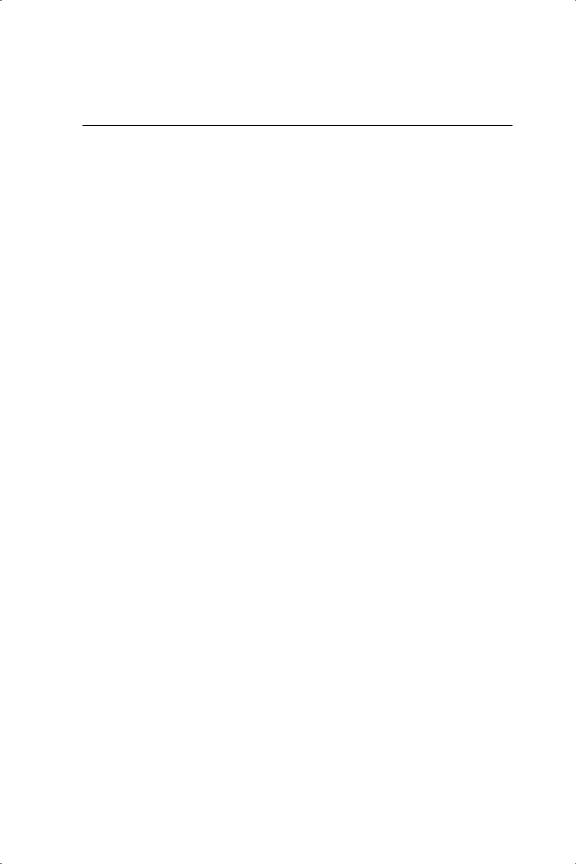
376 |
CHAPTER 8 |
TABLE 8.7. Reaction of 1,3-Butadiene with Deuterium on Metals of Groups 9 to 11: Butadiene Exchange and Deuterium Content of Butenes38,59
|
Butadiene Exchange |
|
|
Initial Ma of Butenes |
|
|
|||
Metal |
|
Ma |
T /K |
|
1-b |
Z -2-b |
E -2-b |
%Db |
|
Co |
0.020 |
374 |
1.0 |
1.4 |
1.4 |
68 |
|
||
Ni |
0.005 |
341 |
1.6 |
1.8 |
1.5 |
65 |
|
||
Cu |
0.042 |
393 |
0.5 |
0.5 |
0.5 |
66 |
|
||
Rh |
0.06 |
373 |
1.8 |
2.0 |
1.9 |
72 |
|
||
Pd |
0.05 |
290 |
1.7 |
1.6 |
2.0 |
72 |
|
||
Pt |
0.01 |
293 |
1.7 |
2.0 |
1.9 |
6.2 |
|
||
|
|
|
|
|
|
|
|
|
|
a M = mean number of D atoms per molecule (for butadiene, values after similar conversions). b Deuterium content of ‘pool’ of atoms, derived from the N-profile procedure.
by quoting detailed deuterobutene distributions; they have been fully analysed by the N-profile procedure, which yields the deuterium content D of the ‘pool’ of atoms with which N positions in the hydrocarbon have equilibrated. We proceed to consider the mechanisms that have been advanced to account for the calculated profiles.
Before entering into detail, a few remarks of a somewhat philosophical nature are in order. Mechanistic statements for C4 species are inevitably more complex than those for C2 and C3 species, and structures become possible that have no analogues in the smaller ones (Section 4.4). In particular, some of them may be represented in alternative but operationally equivalent ways, the choice between them only being resolved by reference to the relevant organometallic chemistry. For example, the adsorbed state of the butadiene molecule may be formulated (see Figure 8.6) as either di-σ or π (II) or tetra-σ or di-π (I) or as hybrid π3σ (IA,IIIB) or π4 (IIIA) forms. [Note, the subscript to π shows the number of carbon atoms in the π-alkenylic bond]. The way in which the reaction proceeds, i.e. the structure of the half-hydrogenated state, then depends on the structure selected for the butadiene. Following precedent, CC bonds interacting with the surface are shown in Figure 8.6 just as π-bonds; di-σ equivalents are not represented, nor are the tri-σ versions of IV and VII, nor is I as π -di-σ.
An additional and very important dimension is provided by the fact that many of the species shown in Figure 8.6 can exist in two different conformations designated syn and anti. This applies to butadiene whether as the di-π forms I and III or the π3σ forms IA and IIIB (IIIA can only adopt the syn form), and to the half-hydrogenated butenyls formed therefrom. Now in the gas phase the anti conformation of butadiene is preferred to the syn by a factor of about 20 at room temperature,14,54 so direct chemisorption into any of these structures (not going via II) automatically favours the former, and this geometry is maintained after the first hydrogen atom is added. The π3-butenyls cannot interconvert, except via IV, V and VII: a temporary detachment of the π -bond from the surface, followed by