
Physics of biomolecules and cells
.pdfM. Dogterom: Polymerization Forces |
209 |
filament is independent of q and given by
Fstall = |
∆G |
= kBT ln |
kon |
· |
(4) |
δ |
ko |
A more mechanistic view on the force generation process is provided by the thermal ratchet model originally introduced by Peskin et al. to describe force generation by actin filaments [6]. In Figure 1B one imagines that assembly takes place in contact with a “barrier” on which a load F is applied. Insertion of new subunits takes place at the contact point between the growing filament and the barrier. In the absence of thermal fluctuations there is no space between these two objects and proteins are physically prevented from attaching to the filament. Thermal fluctuations (e.g., in the form of di usion of the barrier) create temporary gaps that allow new subunits to add themselves to the end of the filament. After such an addition of another subunit, the barrier can no longer di use back to its original location and has thus been “pushed” forward by the assembling filament against the applied load. The o -rate is in this scenario not a ected by the force. When di usion of the barrier over distances of order δ is fast compared to the time between subsequent subunit additions, the rate of assembly is simply the on-rate in the absence of any force or barrier, multiplied by the probability of opening a gap large enough for a new subunit to be added. This probability depends on the energy associated with moving the load F over a distance δ and is given by exp(−δF /kBT ). In this case the force-velocity curve is given by:
V (F ) = δ(kon e−F δ/kBT − ko ) |
(5) |
which is the same result as equation (3) for a force-independent o -rate (q = 1).
In our experiments we aim at measuring the force-velocity behavior for the assembly of cytoskeletal proteins. We hope to learn (by comparison with available models) how the assembly free energy of proteins is converted into mechanical work. But also to find out how large these forces are compared to the forces that are generated by molecular motors that use cytoskeletal filaments as rails and play an equally important role in cellular motility processes. Of course the assembly of cytoskeletal filaments is not as simple as the situation sketched in Figure 1, and care should be taken in comparing experimental results with the simple predictions above. In particular, microtubules consist of (typically) 13 protofilaments forming a hollow tube, assembled from tubulin protein dimers. For an individual microtubule, periods of assembly randomly alternate with periods of disassembly, a process that depends on the hydrolysis of associated GTP molecules [10].

210 |
|
|
|
|
|
|
|
|
|
|
Physics of Bio-Molecules and Cells |
|
|||||||||||||||||||
|
|
|
|
|
|
|
|
|
|
|
|
|
|
|
|
|
|
|
|
|
|
|
|
|
|
|
|
|
|
|
|
|
|
|
|
|
|
|
|
|
|
|
|
|
|
|
|
|
|
|
|
|
|
|
|
|
|
|
|
|
|
|
|
|
|
|
|
|
|
|
|
|
|
|
|
|
|
|
|
|
|
|
|
|
|
|
|
|
|
|
|
|
|
|
|
|
|
|
|
|
|
|
|
|
|
|
|
|
|
|
|
|
|
|
|
|
|
|
|
|
|
|
|
|
|
|
|
|
|
|
|
|
|
|
|
|
|
|
|
|
|
|
|
|
|
|
|
|
|
|
|
|
|
|
|
|
|
|
|
|
|
|
|
|
|
|
|
|
|
|
|
|
|
|
|
|
|
|
|
|
|
|
|
|
|
|
|
|
|
|
|
|
|
|
|
|
|
|
|
|
|
|
|
|
|
|
|
|
|
|
|
|
|
|
|
|
|
|
|
|
|
|
|
|
|
|
|
|
|
|
|
|
|
|
|
|
|
|
|
|
|
|
|
|
|
|
|
|
|
|
|
|
|
|
|
|
|
|
|
|
|
|
|
|
|
|
|
|
|
|
|
|
|
|
|
|
|
|
|
|
|
|
|
|
|
|
|
|
|
|
|
|
|
|
|
|
|
|
|
|
|
|
|
|
|
|
|
|
|
|
|
|
|
|
|
|
|
|
|
|
|
|
|
|
|
|
|
|
|
|
|
|
|
|
|
|
|
|
|
|
|
|
|
|
|
|
|
|
|
|
|
|
|
|
|
|
|
|
|
|
|
|
|
|
|
|
|
|
|
|
|
|
|
|
|
|
|
|
|
|
|
|
|
|
|
|
|
|
|
|
|
|
|
|
|
|
|
|
|
|
|
|
|
|
|
|
|
|
|
|
|
|
|
|
|
|
|
|
|
|
|
|
|
|
|
|
|
|
|
|
|
|
|
|
|
|
|
|
|
|
|
|
|
|
|
|
|
|
|
|
|
|
|
|
|
|
|
|
|
|
|
|
|
|
|
|
|
|
|
|
|
|
|
|
|
|
|
|
|
|
|
|
|
|
|
|
|
|
|
|
|
|
|
|
|
|
|
|
|
|
|
|
|
|
|
|
|
|
|
|
|
|
|
|
|
|
|
|
|
|
|
|
|
|
|
|
|
|
|
|
|
|
|
|
|
|
|
|
|
|
|
|
|
|
|
|
|
|
|
|
|
|
|
|
|
|
|
|
|
|
|
|
|
|
|
|
|
|
|
|
|
|
|
|
|
|
|
|
|
|
|
|
|
|
|
|
|
|
|
|
|
|
|
|
|
|
|
|
|
|
|
|
|
|
|
|
|
Fig. 2. Experiment to measure the force-velocity curve for a single growing microtubule. A) Schematic representation of the experimental set-up (see text). B) DIC microscopy images of a growing microtubule before (left) and after (right) encountering the barrier. The crosses indicate the shape of the microtubule as recognized by our image analysis software. The solid line is the result of fitting this shape to the shape of an elastic rod. The arrow gives the direction of the force as obtained from the fit. Scale bar is 5 µm. C) Contour length (left) and parallel component of the force (right) as a function of time as obtained from the fits. The initial (zero force) growth velocity of this microtubule was around 2.5 µm/min [12].
Figure 2A shows schematically the experimental set-up that we developed to study, quantitatively, the forces generated by single growing microtubules [11, 12]. Short pieces of stabilized microtubules (templates) are biochemically attached to a glass substrate that has been decorated with barriers for growing microtubules. The barriers are lines (15 µm wide, 2 µm high) of silicon-monoxide that were deposited with lithography techniques. When tubulin proteins are added, microtubules grow from the templates,
M. Dogterom: Polymerization Forces |
211 |
some of which encounter the barriers. An important technical detail of these barriers is that they were created with a small “undercut” by briefly etching the substrate in hydrofluoric acid. These undercuts force the microtubule ends to stay in the focal plane of the microscope and prevent them from sliding upwards after reaching the barrier. When microtubules hit the barrier they generally continue to grow. To accommodate the increase in length, two things can happen: either the elongating microtubule end slides laterally along the barrier, giving rise to a modest deflection of the microtubule. Or the microtubule end is hindered in this lateral motion (by an encountered irregularity in the barrier profile) which results in a more dramatic buckling of the microtubule as its growing end pivots around a fixed contact point with the barrier; see Figure 2B. In this last case the elastic restoring force of the buckled microtubule puts a significant load on the microtubule, directly a ecting the further growth of the filament. Using image analysis to obtain the shape of the growing microtubule as a function of time and fitting the obtained curves to the shape of an elastic rod, both the magnitude and the direction of the force acting on the microtubule end can be determined [13]. Also the increase in microtubule length and thus the growth velocity can be derived from these fits (Fig. 2C). Calibration is provided by an independent measurement of the flexural rigidity and the forces one finds are close to the critical buckling force of a homogeneous elastic rod, given the appropriate boundary conditions.
Note that compared to the situation in Figure 1, it is in this case not the barrier that is fluctuating but the position of the assembling filament end itself. In addition the load is not applied externally, but caused by the elastic deformation of the filament itself. This however does not change the ratchet behavior, as long as the fluctuations in the gap size are su ciently fast and the applied load is not itself dependent on the gap size.
Figure 3 shows a force-velocity curve for microtubules obtained after averaging many data such as shown in Figure 2. As said before one should take care in comparing these experimental results with the simple Brownian ratchet model described above. A microtubule consists of 13 laterally connected protofilaments forming a hollow tube, instead of a single linear filament, and the geometrical details of the growth process are not well known. (To make matters worse, electron microscopy studies of microtubules have suggested that growing ends consist of sheet-like structures that close into hollow tubes during the assembly process [14].) In addition, there is the possibility that the hydrolysis of GTP, responsible for the occasional switching to a shrinking state, should be taken into account to understand the response of the growth process to force. In this case the stall force may no longer be simply connected to the free energy associated with tubulin assembly.
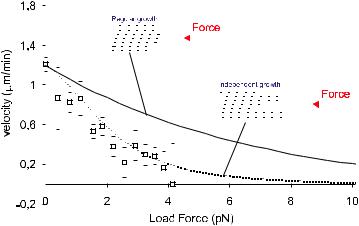
212 |
|
|
|
|
Physics of Bio-Molecules and Cells |
|
|
|
|||||||||||||||||||||||||||||
|
|
|
|
|
|
|
|
|
|
|
|
|
|
|
|
|
|
|
|
|
|
|
|
|
|
|
|
|
|
|
|
|
|
|
|
|
|
|
|
|
|
|
|
|
|
|
|
|
|
|
|
|
|
|
|
|
|
|
|
|
|
|
|
|
|
|
|
|
|
|
|
|
|
|
|
|
|
|
|
|
|
|
|
|
|
|
|
|
|
|
|
|
|
|
|
|
|
|
|
|
|
|
|
|
|
|
|
|
|
|
|
|
|
|
|
|
|
|
|
|
|
|
|
|
|
|
|
|
|
|
|
|
|
|
|
|
|
|
|
|
|
|
|
|
|
|
|
|
|
|
|
|
|
|
|
|
|
|
|
|
|
|
|
|
|
|
|
|
|
|
|
|
|
|
|
|
|
|
|
|
|
|
|
|
|
|
|
|
|
|
|
|
|
|
|
|
|
|
|
|
|
|
|
|
|
|
|
|
|
|
|
|
|
|
|
|
|
|
|
|
|
|
|
|
|
|
|
|
|
|
|
|
|
|
|
|
|
|
|
|
|
|
|
|
|
|
|
|
|
|
|
|
|
|
|
|
|
|
|
|
|
|
|
|
|
|
|
|
|
|
|
|
|
|
|
|
|
|
|
|
|
|
|
|
|
|
|
|
|
|
|
|
|
|
|
|
|
|
|
|
|
|
|
|
|
|
|
|
|
|
|
|
|
|
|
|
|
|
|
|
|
|
|
|
|
|
|
|
|
|
|
|
|
|
|
|
|
|
|
|
|
|
|
|
|
|
|
|
|
|
|
|
|
|
|
|
|
|
|
|
|
|
|
|
|
|
|
|
|
|
|
|
|
|
|
|
|
|
|
|
|
|
|
|
|
|
|
|
|
|
|
|
|
|
|
|
|
|
|
|
|
|
|
|
|
|
|
|
|
|
|
|
|
|
|
|
|
|
|
|
|
|
|
|
|
|
|
|
|
|
|
|
|
|
|
|
|
|
|
|
|
|
|
|
|
|
|
|
|
|
|
|
|
|
|
|
|
|
|
|
|
|
|
|
|
|
|
|
|
|
|
|
|
|
|
|
|
|
|
|
|
|
|
|
|
|
|
|
|
|
|
|
|
|
|
|
|
|
|
|
|
|
|
|
|
|
|
|
|
|
|
|
|
|
|
|
|
|
|
|
|
|
|
|
|
|
|
|
|
|
|
|
|
|
|
|
|
|
|
|
|
|
|
|
|
|
|
|
|
|
|
|
|
|
|
|
|
|
|
|
|
|
|
|
|
|
|
|
|
|
|
|
|
|
|
|
|
|
|
|
|
|
|
|
|
|
|
|
|
|
|
|
|
|
|
|
|
|
|
|
|
|
|
|
|
|
|
|
|
|
|
|
|
|
|
|
|
|
|
|
|
|
|
|
|
|
|
|
|
|
|
|
|
|
|
|
|
|
|
|
|
|
|
|
|
|
|
|
|
|
|
|
|
|
|
|
|
|
|
|
|
|
|
|
|
|
|
|
|
|
|
|
|
|
|
|
|
|
|
|
|
|
|
|
|
|
|
|
|
|
|
|
|
|
|
|
|
|
|
|
|
|
|
|
|
|
|
|
|
|
|
|
|
|
|
|
|
|
|
|
|
|
|
|
|
|
|
|
|
|
|
|
|
|
|
|
|
|
|
|
|
|
|
|
|
|
|
|
|
|
|
|
|
|
|
|
|
|
|
|
|
|
|
|
|
|
|
|
|
|
|
|
|
|
|
|
|
|
|
|
|
|
|
|
|
|
|
|
|
|
|
|
|
|
|
|
|
|
|
|
|
|
|
|
|
|
|
|
|
|
|
|
|
|
|
|
|
|
|
|
|
|
|
|
|
|
|
|
|
|
|
|
|
|
|
|
|
|
|
|
|
|
|
|
|
|
|
|
|
|
|
|
|
|
|
|
|
|
|
|
|
|
|
|
|
|
|
|
|
|
|
|
|
|
|
|
|
|
|
|
|
|
|
|
|
|
|
|
|
|
|
|
|
|
|
|
|
|
|
|
|
|
|
|
|
|
|
|
|
|
|
Fig. 3. Force-velocity curve for the growth of single microtubules [11]. Under these conditions the initial (zero force) growth velocity was around 1.2 µm/min. The solid line is predicted by a regular assembly process; the dotted line is predicted by a model that assumes 13 independently growing filaments. For simplicity only 6 protofilaments are shown in these drawings. In both cases the stall force is arbitrarily set to 18.5 piconewton.
Given what is known about the structure of microtubules, it is tempting to try to include the geometrical details of a growing microtubule into a thermal ratchet model. A simplistic generalization of the original ratchet model describing the growth process of a polymer consisting of two filaments (as is the case for actin) certainly does not fit the available data [11]. In this case it is assumed that the size of the gap needed to insert each new subunit is equal to the added microtubule length per dimer: δ/n, where n is the number of filaments in the polymer (inset Fig. 3; regular growth). Mogilner and Oster therefore generalized the ratchet model described above in a di erent way [15]. They assumed 13 laterally connected, independently growing ratchets, initially arranged as a staircase with subsequent shifts equal to one 13th of the subunit size, with the longest filament in contact with the barrier at any time. Growth of any particular filament requires a fluctuation of the barrier large enough for that filament to insert a new subunit, which thus becomes a function of the distance of the end of that filament to the barrier (inset Fig. 3; independent growth). Through numerical solutions as well as simulations of this model the steady state distribution of filament-barrier distances can be determined as a function of applied force and with this the average growth rate can be calculated. The outcome fits
M. Dogterom: Polymerization Forces |
213 |
the available experimental data surprisingly well. The fit is however very insensitive to the only free parameter in this model: the stall force (i.e. the ratio between the bare onand o -rates, given that their di erence is fixed by a measurement of the growth velocity at zero force), and no conclusions about the stall force can be made [16]. Even though this model makes a lot of implicit assumptions and there is no reason to insist on its details, the outcome of the comparison with the experimental data may in fact suggest that the end of a growing microtubule (under these conditions) looks more like a irregular pointed structure than a blunt end. More data, taken at di erent initial growth velocities, should allow us in the future to test this as well as other hypotheses.
To be able to compare between di erent possible models (including the ones discussed here) we would also like to be able to measure the stall force directly. The buckling technique does however not allow for such a measurement. Due to the geometry of the experiment the force on the microtubule end never increases after the buckling of the microtubule has started, and in fact decreases during the course of the experiment (due to the strong dependence of the critical buckling force on the filament length: Fcrit L−2). Microtubules that are attached relatively close to the barrier stop growing as soon as they encounter the barrier, apparently because the force needed to overcome their critical buckling force is too large. In these cases we have no direct way of measuring the force applied (although estimates can be made). Currently we are therefore working on a second experimental set-up based on optical tweezers techniques [17]. In this setup the microtubule template is attached to two micron-sized silica spheres. These beads are each held in an optical trap orienting the growth direction of the microtubule towards a barrier similar to the one used in the previous experiment. In this case the distance to the barrier should ideally be chosen such that the force needed to buckle the microtubule is larger than the expected stall force. In response to the growth of the microtubule the beads will move with respect to the centers of the optical traps, thereby linearly increasing the force on the growing microtubule end until growth stops. An independent calibration of the trap sti ness will then give a direct measure of the force applied [18].
From a biological point of view, the microscopic details of forces generated in contact with an artificial glass barrier may not be all that interesting. What will be important in the future is to repeat these types of experiments with barriers consisting of chromosomes, kinetochore complexes, or simple motor-coated surfaces. Comparison with our current experiments may reveal important hints as to how molecular growth details and the force generating process are a ected or even regulated by interaction with these specific barriers.
214 |
Physics of Bio-Molecules and Cells |
I would like to thank my current and previous collaborators on this project: Bernie Yurke, Marcel Janson, Sander van Doorn, Catalin Tanase, Bela Mulder, Mahilde de Dood and Jacob Kerssemakers.
References
[1]B. Alberts, A. Johnson, J. Lewis, M. Ra , K. Roberts and P. Walter, Molecular Biology of the Cell (Garland Publishing, New York, London, 2002).
[2]D. Bray, Cell Movements: from Molecules to Motility (Garland, New York, 2000).
[3]L.A. Cameron, P.A. Giardini, F.S. Soo and J.A. Theriot, Nature Rev. Mol. Cell Biol. 1 (2000) 110-119.
[4]D. Pantaloni, C.L. Clainche and M.-F. Carlier, Science 292 (2001) 1502-1506.
[5]S. Inou´e and E.D. Salmon, Mol. Biol. Cell 6 (1995) 1619-1640.
[6]C.S. Peskin, G.M. Odell and G.F. Oster, Biophys. J. 65 (1993) 316-324.
[7]J.A. Theriot, Tra c 1 (2000) 19-28.
[8]M. Dogterom, M.E. Janson, C. Faivre-Moskalenko, A. van der Horst, J.W.J. Kerssemakers, C. Tanase and B.M. Mulder, Appl. Phys. A 75 (2002) 331-336.
[9]T.L. Hill, Linear Aggregation Theory in Cell Biology (Springer-Verlag, New York, Berlin, Heidelberg, 1987).
[10]A. Desai and T.J. Mitchison, Annu. Rev. Cell Dev. Biol. 13 (1997) 83-117.
[11]M. Dogterom and B. Yurke, Science 278 (1997) 856-860.
[12]M.E. Janson and M. Dogterom, in preparation.
[13]F. Gittes, E. Meyhofer, S. Baek and J. Howard, Biophys. J. 70 (1996) 418-429.
[14]D. Chr´etien, S.D. Fuller and E. Karsenti, J. Cell Biol. 129 (1995) 1311-1328.
[15]A. Mogilner and G. Oster, Eur. Biophys. J. 28 (1999) 235-242.
[16]G.S. van Doorn, C. Tanase, B.M. Mulder and M. Dogterom, Eur. Biophys. J. 29 (2000) 2-6.
[17]J.W.J. Kerssemakers, M.E. Janson, A. van der Horst and M. Dogterom, Biophys. J. 82 (2002) 415a.
[18]K. Visscher, S.P. Gross and S.M. Block, IEEE J. Sel. Topics Quant. Elect. 2 (1996) 1066-1076.

COURSE 5
THE PHYSICS OF LISTERIA PROPULSION
J. PROST
Institut Curie, Section de Recherche, UMR 168 du CNRS/IC Physico-Chimie Curie, 26 rue d’Ulm, 75248 Paris Cedex 05, France

Contents
1 |
Introduction |
217 |
|
2 |
A genuine gel |
218 |
|
|
2.1 |
A little chemistry . . . . . . . . . . . . . . . . . . . . . . . . . . . . |
218 |
|
2.2 |
Elastic behaviour . . . . . . . . . . . . . . . . . . . . . . . . . . . . |
220 |
3 |
Hydrodynamics and mechanics |
220 |
|
|
3.1 |
Motion in the laboratory frame . . . . . . . . . . . . . . . . . . . . |
220 |
|
3.2 |
Propulsion and steady velocity regimes . . . . . . . . . . . . . . . . |
221 |
|
3.3 |
Gel/bacterium friction and saltatory behaviour . . . . . . . . . . . |
223 |
4 |
Biomimetic approach |
225 |
|
|
4.1 |
A spherical Listeria . . . . . . . . . . . . . . . . . . . . . . . . . . . |
225 |
|
4.2 |
Spherical symmetry . . . . . . . . . . . . . . . . . . . . . . . . . . |
226 |
|
4.3 |
Steady state . . . . . . . . . . . . . . . . . . . . . . . . . . . . . . . |
227 |
|
4.4 |
Growth with spherical symmetry . . . . . . . . . . . . . . . . . . . |
229 |
|
4.5 |
Symmetry breaking . . . . . . . . . . . . . . . . . . . . . . . . . . . |
229 |
|
4.6 |
Limitations of the approach and possible improvements . . . . . . |
231 |
5 |
Conclusion |
234 |

THE PHYSICS OF LISTERIA PROPULSION
J. Prost
1 Introduction
Listeria is a pathogenic bacterium, which can be dangerous for immune deficient individuals. It can be found almost everywhere, in particular in food such as soft cheese and smoked salmon. After ingestion, it is able to penetrate in the cellular system where it moves from cell to cell and divides on average every twenty minutes. The reason why it can move from cell to cell is that it develops a comet-like tail (Fig. 1), which pushes the bacterium forward and deforms the plasma membrane until it invades the neighbouring cell. Since it is inside the cellular system it is hard to be detected by the immune system. In order to understand the Listeria propulsion mechanism, a particularly intense scientific activity has been developed over these last years [1,2]. Why should one be particularly interested in this problem? The reason is that Listeria motility is due to the polymerisation and crosslinking of an actin gel (i.e. the comet) just like eukaryotic cell motility is due to the polymerisation of actin in the cell lamellipodium. It is then believed that learning something on Listeria is useful for understanding eukaryotic cells as well. Of course studying Listeria does not avoid studying eukaryotic cells since there are many more aspects to eukaryotic motility than to Listeria motility [3] (like adhesion, molecular motors etc.). Yet this allows us to select one aspect, namely actin polymerisation and cross-linking, in geometrical conditions, which are much simpler than those of eukaryotic cells since the process is exterior to the bacterium. One can in particular use cell extracts to perform in vitro experiments. Yet a priori simpler than eukaryotic cell motility, Listeria motility has its mysteries: in particular a mutant has been observed to move by a succession of jumps followed by periods during which the bacteria is essentially immobile [4] (Fig. 2). During the waiting period the gel grows around the whole bacterium producing some kind of sheath. Eventually the bacterium gets expelled from the sheath, hence the jerky motion. In the following I give a few guidelines for thinking about the physics of the propulsion mechanism, and show that it is essentially a continuum mechanics problem with very unusual boundary conditions.
c EDP Sciences, Springer-Verlag 2002

218 |
Physics of Bio-Molecules and Cells |
Fig. 1. Example of a wild type Listeria and its homogeneous comet.
2 A genuine gel
2.1 A little chemistry
Before getting to the characterisation of the gel, it is necessary to give a few tips concerning the biochemistry of the polymerisation process. First, it is now well established that in order to observe the formation of a comet, a particular enzyme called ActA must be present on the surface of the bacterium. In vitro experiments can be made by placing bacteria in cell extracts, or in reconstituted extracts. The presence of ActA is necessary but not su cient for getting a polymerisation process comparable to the one observed in vivo. Actin filaments are polar: they have two fundamentally di erent extremities. One, called barbed (or plus) end, can polymerise while the other, called pointed (or minus), can simultaneously de-polymerise. Of course this can only happen if energy is constantly fed into the system and this is done by hydrolysing ATP in ADP. Phosphorylated monomers polymerise at the barbed end, while dephosphorylated monomers de-polymerise at the pointed end. In the absence of any other protein the polymerisation