
Matta, Boyd. The quantum theory of atoms in molecules
.pdf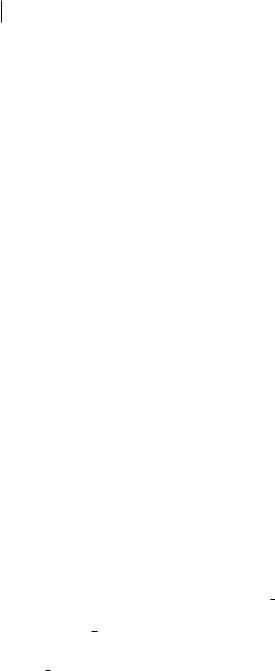
62 3 Atomic Response Properties
distribution within the nuclear-centered atom, is similar to that for the molecule as a whole. The transfer contribution associated with a bonded group to an atomic response property is given by the weighted translation vector from the atom’s nucleus to the corresponding bond critical point, the weight being the bonded group’s contribution to the appropriate ‘‘null’’ molecular property. This method not only provides transferable atomic and group response properties consistent with additivity schemes developed from experimental data [3–10], but is also quite intuitive. For atoms in one or more rings, however, ambiguities may arise in the definition of a bonded group. It is, therefore, necessary to restate the transfer contributions to an atomic response property more generally, in terms of atomic bond contributions. One well defined and computationally straightforward method for defining such atomic bond contributions to any ‘‘null’’ properties and for any molecular system, including those with rings and cages, is described in this chapter. In the absence of rings and cages, this more general method reduces to the original method of Bader et al. [2–10]. Using this method, expressions for atomic contributions to electric dipole moments, electric static dipole polarizabilities, infrared vibrational absorption intensities, nuclear virial energies, and magnetizabilities are presented in some detail, with some illustrative numerical examples.
Although inclusion of nuclear virial energies in a list of response properties may seem out of place, a discussion of these is included because many molecular response properties of interest explicitly involve energy derivatives with respect to nuclear positions (e.g. vibrational frequencies) or involve non-stationary point geometries (e.g., vertical transition energies) and, therefore, a well defined atomic contribution to the total molecular energy (i.e. electronic energy plus nuclear virial energy) is necessary to determine such atomic response properties. In addition, a working definition of an atomic contribution to the molecular nuclear virial energy seems to require overcoming origin-dependence issues similar to those encountered with the other response properties described here.
3.2
Apparent Origin-dependence of Some Atomic Response Properties
When the electric dipole moment m of a molecule is simply partitioned into atomic contributions [2], the atomic contribution, mðWÞ, for atom W consists of an origin-independent ‘‘polarization’’ term, mpðWÞ, and an origin-dependent ‘‘charge transfer’’ term, mcðWÞ:
ð
mðWÞ ¼ ½r RW&rðrÞ dr þ ½RW R0&QðWÞ ¼ mpðWÞ þ mcðWÞ ð1Þ
W
where QðWÞ is the net charge of atom W, RW is the position vector of atom W, and R0 is the arbitrary origin of the molecular coordinate system. Note that underlines are used here to indicate origin-dependent terms.

3.2 Apparent Origin-dependence of Some Atomic Response Properties 63
Because mcðWÞ is dependent on R0 (unless QðWÞ is zero), mðWÞ as defined in Eq.
(1) is not generally meaningful, just as m itself is not usually meaningful for charged molecules.
Laidig and Bader [3] first showed that for neutral molecules, a more sophisticated definition for the ‘‘charge transfer’’ atomic contribution can be used, mcðWÞ, one which is both origin-independent and physically reasonable:
NbðWÞ |
|
X1 |
ð2Þ |
mcðWÞ ¼ ½RbðWjLÞ RW&QðLWGÞ |
|
L¼ |
|
In this expression NbðWÞ is the number of bond critical points connected to the nucleus of atom W, RbðWjLÞ is the position vector of the bond critical point between atom W and atom L, and QðLWGÞ is the net charge of the group LWG that is bonded to atom W via atom L. For example, in the methanol molecule RbðCjOÞ is the position vector of the bond critical point between the C and O atoms and QðOCGÞ is the net charge of the OH group.
Formulas analogous to Eq. (2) have also been used to partition polarizability tensors [3, 4], magnetizability tensors [5–7], and electronic transition moments [8] of molecules into origin-independent atomic and group contributions.
For molecular structures in which atoms W and L are bonded within one or more rings, however, QðLWGÞ is usually not well defined (the exception being symmetrically equivalent bonds connecting the atom within the ring or rings) because the group LWG to which atom L belongs has at least one other bond to atom W, say via atom L0, and it is thus ambiguous which portion of the group containing both L and L0 is defined as LWG and which is defined as L0WG. While for atoms in single rings one may attempt to use the well defined ring critical point instead of the two corresponding bond critical points in Eq. (2), for atoms in more than one ring this will not work and one is again left with an ambiguous definition for LWG.
Contrary to what has been stated previously [6, 8–10], for isolated molecules this problem cannot be solved by using Gauss’ law to express QðLWGÞ as the electric field flux through the interatomic surface between atom L and atom W.
mcðWÞ 0 ð 1=4pÞ |
NbðWÞ½RbðWjLÞ RW& þ dSðLjW; rsÞ EðrsÞ |
|
|
|
X1 |
|
|
|
L |
¼ |
|
|
|
|
|
(for isolated molecules) |
ð3Þ |
The inequality in Eq. (3) ultimately arises because the net charge of an atom in a molecule cannot be solely expressed in terms of electric field fluxes through its interatomic surfaces unless the atom is finite in size, i.e. unless the union of its interatomic surfaces defines a surface completely enclosing a finite volume for the atom:

64 3 Atomic Response Properties
QðWÞ ¼ ð 1=4pÞ þ |
dSðWjInf; rsÞ EðrsÞ |
|
|
þ ð 1=4pÞ |
NbðWÞ þ dSðWjL; rsÞ EðrsÞ |
ð4Þ |
|
|
X1 |
|
|
|
L |
¼ |
|
|
|
|
If a portion SðWjInf Þ of an atom’s bounding surface extends to infinity, the first term on the RHS of Eq. (4) will usually be non-zero. Unlike local property density functions (e.g., the electron density and its derivatives, the stress tensor, the current density, etc.), which decay exponentially far from a molecule, the electric field is a non-local function that decays only as r 2 far from a molecule. Because a surface grows in area as r2, the contribution to the net electric field flux through the infinite portions of an atomic surface will not vanish.
To extend Eq. (2) to systems with rings and cages it seems useful, if not necessary, to dispense with the notion of the bonded group LWG and simply restate Eq.
(2) more simply, in terms of bond contributions:
NbðWÞ |
|
X1 |
ð5Þ |
mcðWÞ ¼ ½RW RbðWjLÞ&QðWjLÞ |
|
L¼ |
|
where QðWjLÞ is now defined as the contribution from the directed bond between atoms W and L to the net charge of atom W. The problem is then to be able to uniquely determine a set of such bond charges QðWjLÞ in any molecule to enable use of Eq. (5). In the absence of a ring containing W and L, QðWjLÞ should equal QðLWGÞ, so Eq. (2) is recovered in those instances.
The situation is similar for atomic polarizability, magnetizability and other response properties.
In the following section, a method of partitioning any ‘‘null’’ (zero value) molecular property h of any molecular structure into unique bond contributions, hðWjLÞ, is described.
3.3
Bond Contributions to ‘‘Null’’ Molecular Properties
Consider any ‘‘null’’ (zero value) property h of a molecule. Examples of h are:
the net charge, Q, of a neutral molecule
the change in net charge, dQ, of a molecule due to a perturbation such as an electric field or nuclear displacement
the net current vector, hJi, induced in a molecule by an external magnetic field (when the continuity equation is satisfied)
the net Ehrenfest force, hFEi, acting on the electrons
the energy-gradient-based force, GA, acting on a nucleus A at an equilibrium or other stationary point geometry

3.3 Bond Contributions to ‘‘Null’’ Molecular Properties 65
the Hellman–Feynman electrostatic force, FA, acting on a nucleus A at an equilibrium geometry or other stationary point geometry (when the Hellman–Feynman electrostatic theorem is satisfied)
the sum of the Hellman–Feynman electrostatic forces, SðFAÞ, acting on the nuclei at any geometry (when the Hellman– Feynman electrostatic theorem is satisfied)
the sum of the energy-gradient-based forces, SðGAÞ, acting on the nuclei at any geometry
Although such properties vanish for molecules, their atomic contributions hðWÞ and corresponding bond contributions hðWjLÞ will not typically vanish. The contributions hðWÞ and hðWjLÞ may be of interest directly and/or they may appear in expressions for other atomic properties that are of interest. Equations (2) and (5) are examples of the latter.
While the atomic property hðWÞ is typically well defined and easily calculated by simple partitioning of h (as in each of the cases listed above), a method for calculating the corresponding bond contributions hðWjLÞ has not been previously defined in a general and computationally convenient way.
Considering a molecule with Na atoms, h is a sum of Na atomic contributions hðWÞ:
Na
X
h ¼ hðWÞ ¼ 0 |
ð6Þ |
W¼1 |
|
Using QTAIM [1] to uniquely identify the bonds, rings and cages in a molecule, each atomic contribution hðWÞ to h can be expressed as a sum of bond contributions hðWjLÞ:
NbðWÞ
X
hðWÞ ¼ |
hðWjLÞ |
ð7Þ |
L¼1
where NbðWÞ is the number of bond critical points to atom W and hðWjLÞ is the contribution to hðWÞ from the directed bond between atoms W and atom L. To uniquely solve for all of the 2Nb bond contributions in a molecule, the following constraint (meaningful only for ‘‘null’’ molecular properties) is used for each bond:
hðWjLÞ þ hðLjWÞ ¼ 0 |
ð8Þ |
In the absence of rings, the set of Na þ Nb equations defined by Eqs (7) and (8) is su cient to uniquely determine the 2Nb ¼ Na þ Nb 1 bond contributions because one of the equations is redundant, due to the constraint of Eq. (6). If the

66 3 Atomic Response Properties
molecule contains one or more rings, however, an additional constraint is necessary for each ring. The following constraint is proposed here:
NaðRÞ
X
hðWjW þ 1Þ ¼ 0 |
ð9Þ |
W¼1
where NaðRÞ is the number of atoms in ring R and hðWjW þ 1Þ is the contribution from the bond between the Wth atom in the ring and the next atom in the ring. Note that the counting is done one-way (clockwise or counterclockwise) but that either one-way sum is equivalent, because of Eq. (8). Note, however, that Eq. (9) itself does not follow from Eq. (8).
Equation (9) states that the one-way sum of the bond contributions from a ring to a ‘‘null’’ molecular property is necessarily zero, because a ring is a closed circuit of bonds. For a molecule with Nr rings, Eqs (7)–(9) constitute a set of Na þ Nb þ Nr linear equations involving 2Nb unknowns. There is, however, one redundant equation, because of the constraint of Eq. (6) and also one redundant ring equation for each cage. For a molecule with Nc cages there are, therefore, Na þ Nb þ Nr 1 Nc linearly independent equations. Using the Poincare´– Hopf relationship between Na, Nb, Nr, and Nc [1]:
Number of independent equations ¼ Na þ Nb þ Nr 1 Nc ¼ 2Nb
¼ number of bond contributions |
ð10Þ |
Thus, the set of linear equations defined by Eqs (7)–(9) has a unique solution for any structure and any ‘‘null’’ property and can easily be solved without manual e ort using a linear equation solver such as the DGELSS routine of LAPACK [11], which enables apparently (but only apparently) overdetermined sets of equations as input.
Figures 3.1–3.8 show the bond contributions hðWjLÞ to a generic ‘‘null’’ molecular property h for some generic molecular structures, in terms of the atomic contributions to h. Although an understanding, or picture, of the bond contributions hðWjLÞ in terms of atomic contributions is not necessary to use them, occasionally such pictures may be helpful for interpretation.
The result obtained from Eqs (7)–(9) for a bond contribution hðWjLÞ in a ring system is equivalent to the result of averaging the corresponding bond contribu-
AaaB
hðAjBÞ ¼ hðAÞ hðBjAÞ ¼ hðBÞ
Fig. 3.1 Bond contributions to h in a diatomic molecule.

3.3 Bond Contributions to ‘‘Null’’ Molecular Properties 67
AaaBaaC
hðAjBÞ ¼ hðAÞ hðBjAÞ ¼ hðAÞ hðBjCÞ ¼ hðCÞ hðCjBÞ ¼ hðCÞ
Fig. 3.2 Bond Contributions to h in a triatomic chain molecule.
A
= n
BaaC
hðAjBÞ ¼ ð1=3Þ½2hðBÞ þ hðCÞ& hðAjCÞ ¼ ð1=3Þ½2hðCÞ þ hðBÞ& hðBjAÞ ¼ ð1=3Þ½2hðAÞ þ hðCÞ& hðBjCÞ ¼ ð1=3Þ½2hðCÞ þ hðAÞ& hðCjAÞ ¼ ð1=3Þ½2hðAÞ þ hðBÞ& hðCjBÞ ¼ ð1=3Þ½2hðBÞ þ hðAÞ&
Fig. 3.3 Bond contributions to h in a triatomic ring molecule.
A
= n
BaaCaaD
hðAjBÞ ¼ ð1=3Þ½2hðBÞ þ hðCÞ þ hðDÞ& hðAjCÞ ¼ ð1=3Þ½2hðCÞ þ 2hðDÞ þ hðBÞ& hðBjAÞ ¼ ð1=3Þ½2hðAÞ þ hðCÞ þ hðDÞ& hðBjCÞ ¼ ð1=3Þ½2hðCÞ þ 2hðDÞ þ hðAÞ& hðCjAÞ ¼ ð1=3Þ½2hðAÞ þ hðBÞ&
hðCjBÞ ¼ ð1=3Þ½2hðBÞ þ hðAÞ& hðCjDÞ ¼ hðDÞ
hðDjCÞ ¼ hðDÞ
Fig. 3.4 Bond contributions to h in a branched triatomic ring molecule.

683 Atomic Response Properties
AaaBaaCaaD
hðAjBÞ ¼ hðAÞ hðBjAÞ ¼ hðAÞ
hðBjCÞ ¼ hðCÞ hðDÞ hðCjBÞ ¼ hðAÞ hðBÞ hðCjDÞ ¼ hðDÞ hðDjCÞ ¼ hðDÞ
Fig. 3.5 Bond contributions to h in a tetraatomic chain molecule.
C
=
AaaB n
D
hðAjBÞ ¼ hðAÞ hðBjAÞ ¼ hðAÞ hðBjCÞ ¼ hðCÞ hðBjDÞ ¼ hðDÞ hðCjBÞ ¼ hðCÞ
hðDjBÞ ¼ hðDÞ
Fig. 3.6 Bond contributions to h in a tetraatomic branched chain molecule.
tion resulting from every possible way of (artificially) minimally opening the ring (or rings) in which A and B are connected such that they are no longer connected in a ring or rings. Figures 3.9 and 3.10 illustrate this for a triatomic ring and a tetraatomic bicyclic ring.
Although a procedure for determining hðWjLÞ on the basis of such pictures would obviously be tedious, the pictures do provide some insight into the meaning of the ring constraint defined by Eq. (9).
As shown in Section 3.2 for electric dipole moments, one of the uses of the decomposition of ‘‘null’’ molecular properties into bond contributions is that apparently origin-dependent atomic contributions to other molecular properties, such as electric dipole moments, electric polarizabilities, magnetizabilities, infrared vibrational absorption intensities, and nuclear virial energies, can be defined in an origin-independent and physically reasonable manner.

3.3 Bond Contributions to ‘‘Null’’ Molecular Properties 69
AaaD
j j
BaaC
hðAjBÞ ¼ ð1=4Þ½3hðBÞ þ 2hðCÞ þ hðDÞ& hðAjDÞ ¼ ð1=4Þ½3hðDÞ þ 2hðCÞ þ hðBÞ& hðBjAÞ ¼ ð1=4Þ½3hðAÞ þ 2hðDÞ þ hðCÞ& hðBjCÞ ¼ ð1=4Þ½3hðCÞ þ 2hðDÞ þ hðAÞ& hðCjBÞ ¼ ð1=4Þ½3hðBÞ þ 2hðAÞ þ hðDÞ& hðCjDÞ ¼ ð1=4Þ½3hðDÞ þ 2hðAÞ þ hðBÞ& hðDjAÞ ¼ ð1=4Þ½3hðAÞ þ 2hðBÞ þ hðCÞ& hðDjCÞ ¼ ð1=4Þ½3hðCÞ þ 2hðBÞ þ hðAÞ&
Fig. 3.7 Bond contributions to h in a tetraatomic ring molecule.
A
= n
BaaC n =
D
hðAjBÞ ¼ ð1=8Þ½5hðBÞ þ 3hðCÞ þ 4hðDÞ& hðAjCÞ ¼ ð1=8Þ½5hðCÞ þ 3hðBÞ þ 4hðDÞ& hðBjAÞ ¼ ð1=8Þ½5hðAÞ þ 2hðCÞ þ hðDÞ& hðBjCÞ ¼ ð1=4Þ½2hðCÞ þ hðAÞ þ hðDÞ& hðBjDÞ ¼ ð1=4Þ½5hðDÞ þ 2hðCÞ þ hðAÞ& hðCjAÞ ¼ ð1=8Þ½5hðAÞ þ 2hðBÞ þ hðDÞ& hðCjBÞ ¼ ð1=4Þ½2hðBÞ þ hðAÞ þ hðDÞ& hðCjDÞ ¼ ð1=4Þ½5hðDÞ þ 2hðBÞ þ hðAÞ& hðDjBÞ ¼ ð1=8Þ½5hðBÞ þ 3hðCÞ þ 4hðAÞ& hðDjCÞ ¼ ð1=8Þ½5hðCÞ þ 3hðBÞ þ 4hðAÞ&
Fig. 3.8 Bond contributions to h in a tetraatomic bicylic molecule.
70 |
3 Atomic Response Properties |
|
|
|
|
|
|
|
||
|
A |
A |
A |
|
|
A |
|
|
|
|
|
|
|
|
|
|
|
||||
|
= n |
= |
= n |
|
|
n |
|
|
|
|
|
BaaC |
BaaC |
B C |
|
|
BaaC |
|
|
|
|
1 |
2 |
3 |
|
|
4 |
|
|
|
|
|
|
hðAjBÞ1 ¼ ð1=3Þ½hðAjBÞ2 þ hðAjBÞ3 þ hðAjBÞ4& |
|
|
|
|
|||||
|
|
¼ ð1=3Þ½hðAÞ hðBÞ þ 0& ¼ ð1=3Þ½2hðBÞ þ hðCÞ& |
|
|
||||||
|
Fig. 3.9 |
Bond Contributions to h in a Tetraatomic Bicylic Molecule. |
|
|
||||||
|
A |
A |
A |
|
A |
A |
A |
A |
A |
A |
|
= n |
= |
= |
= |
|
= n |
= n |
n |
n |
n |
|
BaaC BaaC B C BaaC B C B C B C BaaC BaaC |
|||||||||
|
n = |
n |
n = |
|
= |
n |
= |
n = |
= |
n |
|
D |
D |
D |
|
D |
D |
D |
D |
D |
D |
1 |
2 |
3 |
|
4 |
5 |
6 |
7 |
8 |
9 |
hðAjBÞ1 ¼ ð1=8Þ½hðAjBÞ2 þ hðAjBÞ3 þ hðAjBÞ4 þ hðAjBÞ5 þ hðAjBÞ6
þ hðAjBÞ7 þ hðAjBÞ8 þ hðAjBÞ9&
¼ð1=8Þ½5hðBÞ þ 3hðCÞ þ 4hðDÞ&
Fig. 3.10 Bond Contributions to h in a Tetraatomic Bicylic Molecule.
It should be emphasized that this method is only applicable to ‘‘null’’ molecular properties, because of the constraints defined in Eqs (6)–(9). A generalization of Eqs (6)–(9) to the decomposition of any molecular property P (‘‘null’’ or ‘‘nonnull’’) into physically reasonable bond contributions might be possible, and would be highly desirable, but is not considered further here except to note that such a method might produce the following formula for PðWjLÞ when W and L are not connected in a ring:
PðWjLÞ ¼ ½PðWÞ=ðP PðWÞÞ&PðLWGÞ |
ð11Þ |
where PðLWGÞ is the net value of P for the group that is bonded to atom W via atom L.
3.4
Bond Contributions to Atomic Charges in Neutral Molecules
Using Eq. (7), the atomic charge QðWÞ of an atom W in a neutral molecule can be expressed in terms of corresponding bond charges QðWjLÞ as:
|
3.5 |
Atomic Contributions to Electric Dipole Moments of Neutral Molecules |
71 |
||||
Table 3.1 |
Symmetrically unique atomic charges and their bond |
|
|
|
|
||
|
|
|
|
||||
contributions in para-nitroaniline. |
|
|
|
|
|
||
|
|
|
|
|
|
|
|
Atom, W |
Q(W) |
Q(WSL1)L1 |
Q(WSL2)L2 |
Q(WSL3)L3 |
|||
|
|
|
|
|
|
|
|
C1 |
þ0.542 |
þ0.468 N2 |
þ0.037 C3 |
þ0.037 C4 |
|||
N2 |
1.309 |
0.468 C1 |
0.421 H9 |
0.421 H9 |
|||
C3 |
þ0.047 |
0.037 C1 |
þ0.066 C5 |
þ0.018 |
H7 |
||
C5 |
þ0.068 |
0.066 C3 |
0.063 H12 |
þ0.197 |
C11 |
||
H7 |
0.018 |
0.018 C3 |
|
|
|
|
|
H10 |
þ0.421 |
þ0.421 N2 |
0.197 C6 |
þ0.706 |
|
|
|
C11 |
þ0.313 |
0.197 C5 |
N14 |
||||
H12 |
þ0.063 |
þ0.063 C5 |
þ0.541 O15 |
þ0.541 |
|
|
|
N14 |
þ0.376 |
0.706 C11 |
O16 |
||||
O15 |
0.541 |
0.541 N14 |
|
|
|
|
|
Total[a] |
þ0.002 |
|
|
|
|
|
|
a The deviation from a total net charge of zero is because of numerical integration errors for the atoms. The maximum atomic integration error, as measured by jLðWÞj, was 0.001 a.u. for N14.
NbðWÞ
X
QðWÞ ¼ |
QðWjLÞ |
ð12Þ |
L¼1
where the set of QðWjLÞ are determined by solving the set of linear equations defined by Eqs (7)–(9). As an example, Table 3.1 shows the set of QðWÞ and QðWjLÞ for each symmetrically unique atom in para-nitroaniline (shown in Fig. 3.11), calculated at HF/6-311þþG(2d,2p)//HF/6-311þþG(2d,2p). In addition to being useful for partitioning electric dipole moments and their derivatives into atomic contributions, such bond charges might find application in their own right, as descriptors in QSAR studies or in the construction of molecular mechanics force fields, for example.
3.5
Atomic Contributions to Electric Dipole Moments of Neutral Molecules
The electric dipole moment, m, of a molecule is given by:
m ¼ ð½r R0&rðrÞ dr þ |
Na |
ZW½RW R0& |
ð13Þ |
|
|
X1 |
|
|
|
|
W |
¼ |
|
|
|
|
|
|
where rðrÞ is the electron density at a point r in real space, ZW is the nuclear charge of atom W, RW is the position vector of nucleus W, and R0 is the arbitrary