
Emerging Tools for Single-Cell Analysis
.pdf98 |
Microfabricated Fluidic Devices for Single-Cell Handling and Analysis |
that is the basis for modern flow cytometry. This is illustrated in Figure 5.1. The sample tube introduces the sample stream into the center of a concentric sheath flow. The sample and sheath flow are then routed through a nozzle. Since the Re number is small (i.e., laminar flow), the fluid streams are compressed into the narrower nozzle opening while maintaining their relative dimensions (i.e., the ratio of sample stream diameter to sheath flow diameter remains constant).
Diffusion (Brownian Motion). While turbulence is not an issue, diffusion between the sheath and core is an important consideration. Once the sample leaves the sample tube, it is free to diffuse with the surrounding sheath flow. This diffusion is governed byx2 2Dt, where x2 is the mean square distance, t is time, and D is the diffusion constant.
The diffusion coefficient scales with the effective size of the particle and can vary over four orders of magnitude between small molecules (~1000 m2/s) and cells (<1 m2/s). One must always be cognizant of diffusion issues when designing and evaluating microfluidic systems. Depending on flow rates and diffusion coefficients, diffusion can play a major role in system performance. The impact of diffusion can sometimes be used in a beneficial way. For example, one can expose cells to a known sheath medium in a controlled way by careful control of sheath location/length (Pinkel and Stovel, 1985).
These and other fluid issues related to biological fluids in microscale devices are discussed by Brody et al. (1996) and Wilding et al. (1994).
Microfabricated Cytometry
Microfabrication and MicroElectroMechanical Systems. Over the last 15 years a new class of miniaturization technologies has emerged that will be loosely grouped here under the term MicroElectroMechanical Systems (MEMS). MEMS is a group of technologies that allow for the creation of mixed electrical, mechanical, chemical, and fluidic systems at the micrometer scale. While initially MEMS grew out of traditional integrated circuit techniques, it has now expanded to become a distinct and separate field encompassing a wide range of fabrication methods and materials. Two recent texts provide a good overview of these technologies (Kovacs, 1998; Madou, 1997). The focus here will be to survey work in MEMS related to flow cytometry and single-cell analysis.
Microfabricated Cytometry Devices. The phenomenon of hydrodynamic orientation of cells (discussed above) and the subsequent development of high-speed cytometry in the 1970s and early 1980s predated the current development of microfluidic systems but in fact is based on many of the same concepts. Sobek et al. (1994) recognized this and demonstrated hydrodynamic focusing and sheath flow in a fused-silica device (see Fig. 5.2). The device was fabricated from fused silica using a single mask layer and wafer-level alignment and bonding. The sample injector dimensions were 250 m high by 280 m wide. Hydrodynamic focusing was maintained for velocities as high as 10 m/s with a corresponding inlet-to-outlet pressure drop of 28 psi.
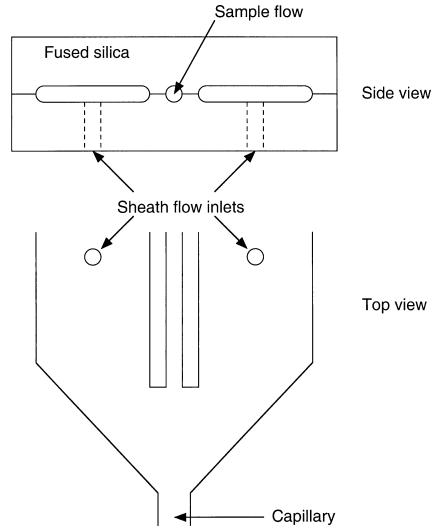
Microfluidics and Cytometry |
99 |
F i g . 5.2. Glass device used to demonstrate the potential of cytometry in microfabricated devices. The sample flow was introduced via a hypodermic needle coupled to the fused-silica sample flow channel. (After Sobek et al., 1994.)
Other demonstrations of simple microfabricated channels designed for cell transport and sorting soon followed. Larsen et al. (1997) presented a microchip Coulter particle counter device. The device was fabricated in silicon with a glass cover. A modified Coulter counter geometry is demonstrated. Five parallel fluid streams are brought together via five separate but parallel channels (see Fig. 5.3). The center three streams are electrolytes and create the traditional sheath flow observed in flow
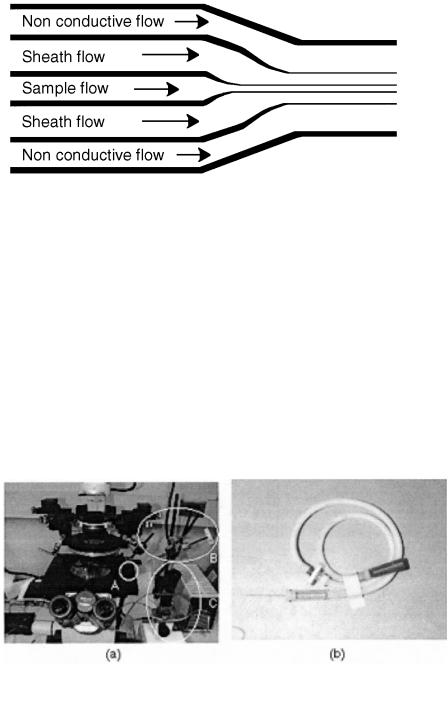
100 |
Microfabricated Fluidic Devices for Single-Cell Handling and Analysis |
F i g . 5.3. Multiple streams can be used to provide dynamic control of the sample stream width. (After Larson et al., 1997.)
cytometry while the other two streams consist of nonconductive fluid and provide the ability to dynamically adjust the effective width of the sheath flow. Since only the middle streams are conductive, the width of those three streams determine the sensitivity of the detection. The published work only demonstrated the fluidic operation of the device; no actual detection results were presented.
Differential counting of granulocytes, lymphocytes, monocytes, red blood cells, and platelets in a silicon flow channel was demonstrated by Altendorf et al. (1997). The device consisted of a single v-groove channel (top width of 20–25 m) (no sheath flow). Laser light scattering (both small and large angle) was the basis of differential counting. A single laser source (638 nm) with two detectors (one for small angle, one for large angle) was used. The sizeand structure-dependent light scattering allows differential counting.
F i g . 5.4. Single-cell handling is typically accomplished with the use of a micromanipulation station and/or mouth pipette. (a) Typical micromanipulation station includes a microscope, pulled pipette tip (A) controlled by a micromanipulator joystick (C). Fluid transport through the pipette is controlled via a precision syringe. (b) Simple mouth pipette is also still commonly used to handle single cells and embryos.

Single-Cell Manipulation and Analysis |
101 |
SINGLE-CELL MANIPULATION AND ANALYSIS
The range of device possibilities that MEMS technologies make possible allows one to envision systems capable of more than just sorting functions. For comparison, we first briefly review the physical tools traditionally used for single-cell manipulation and analysis and then examine current efforts to construct novel microsystems for single-cell study.
Traditional Methods
Current methods for manipulating single cells and embryos have changed little in decades. Researchers still routinely use simple equipment to fabricate custom suction pipettes to hold, probe, and inject single cells and embryos (see Fig. 5.4). For example, Hogan et al. (1994) describe procedures for making holding and injection pipettes for embryo manipulation such as DNA injection and nuclear transplantation. The procedure is the equivalent of micro–glass blowing. One begins with a 1-mm-outside- diameter (OD) glass capillary. Using a microburner (e.g., a small Bunsen burner) or pipette puller (e.g., Sutter Instrument P-87) and a micro forge (e.g., Kramer Scientific), a variety of pipettes can be formed for holding and injection procedures. The inside diameter (ID) of pipettes made using these techniques can be on the order of 1 m or as large as several hundred micrometers. Suction (mechanical or mouth) applied to a holding pipette is used to hold the cell firmly against the pipette tip, while syringes are used on injection pipettes to obtain and expel minute quantities of sample.
Microfabricated Devices and Systems
We are in the midst of rapid growth in the sophistication and variety of microdevices and systems being built to extend our ability to study life at the cellular level and below. Much of this growth is in the area of gene chips to allow rapid genomic analysis. Examples of these technologies include products by Nanogen and Affymetrix. If one thinks beyond the problem of sequencing the genome to the problem of functional genomics, then one must think beyond planar gene chip designs to systems that allow physical interaction with the cell or molecule of interest.
Mechanical Transport, Sorting, Manipulation and Characterization.
Traditionally, cell deformability has been assessed using either micropipette aspiration or filtration. When one can fabricate physical structures at the scale of the cell or molecule in a very controlled way (i.e., lithography-based microfabrication), one can begin to study the physical interaction and characteristics of single cells and molecules in more quantitative and reproducible ways than is possible with either micropipette aspiration or traditional filtration.
Ogura et al. (1991) developed a microfabricated device for the study of human red blood cell deformability. Pores down to 1 m in diameter were created in a 0.4- m- thick silicon nitride membrane. Cell passage times were measured for cells that had been treated in ways known to affect deformability (temperature, exposure to diamide

102 |
Microfabricated Fluidic Devices for Single-Cell Handling and Analysis |
and glutaraldehyde, and storage conditions). Results demonstrated clearly that the device was capable of differentiating cell treatments via passage time measurements. However, the use of short pore filters is largely unexplored and more study is required.
About the same time two groups began investigating the use of micromachined flow channels for the study of blood rheology and blood cell deformability to mimic the in vivo conditions of blood cells traversing capillaries. Tracey et al. (1991) used Reactive Ion Etching (RIE) to form square (4- m) channels while Kikuchi et al. (1992) utilized anisotropic etching to realize v-groove channels with a top width of 9 m (see Fig. 5.5). Tracey et al. (1995) measured erythrocyte length and velocity for normal erythrocytes to demonstrate their device, but in experiments with artificially hardened cells no subpopulations were found. Kikuchi et al. (1992, 1994) and Kikuchi (1995) performed a series of rheology studies using both red and white blood cells (RBC, WBC) with a variety of treatments, including formyl-L-methionyl-L-leucyl-L-phenylalanine (FMLP) and adenosine diphosphate (ADP). Direct comparisons to Nuclepore filters (Nuclepore, Pleasanton, CA) were performed by measuring the passage times. Flow rates were about 50 times higher in the Nuclepore filters (close to expected based on physical differences between filters and channels). Significant variations between channels were reported due to transient clogging and blocking at the channel entrances.
More recently Austin and co-workers have demonstrated the feasibility of using lithographic arrays for cell sorting (Brody et al., 1995; Bakajin et al., 1998; Carlson et al., 1997). Specifically, they have designed and constructed large microfabricated lattices. A typical lattice contains a variety of channel widths and lengths but in much larger numbers than those of Kikuchi (1992) and Tracy (1991), thus allowing separation of larger numbers of cells. The transport and adhesion properties of human RBCs and WBCs have been studied, and the ability to efficiently separate out the WBCs has been demonstrated (see Fig. 5.6).
Glasgow et al. (1998) have developed a system for the transport and precise positioning of single mammalian embryos. The embryos (approximately 120 m diame-
F i g . 5.5. (a) Simple pores can be fabricated with precise control of pore size. Single cells can then be forced through the pores enabling cell mechanics studies. (b) To mimic human capillaries, microchannels can be fabricated. The top illustrates the channel shape used by Kikuchi et al. (1992), while the bottom shows the shape used by Tracy et al. (1995).
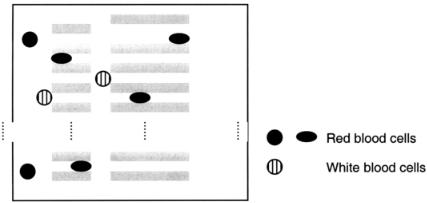
Single-Cell Manipulation and Analysis |
103 |
F i g . 5.6. Constrictions in the lattice are similar to those shown in Figure 5 b, bottom. The WBCs tend to get caught at the entrance to the channels rather than within the channels. (After Carlson et al., 1997.)
ter) are on the same order of size as the channels (approximately 200 m). The similar scale of object and channel causes the embryos to roll and slip along the channel bottoms. The device also incorporates physical restrictions that allow the embryos to be positioned in precise locations (analysis stations) and then to be easily moved to another location or directed to different outlets (see Fig. 5.7). The integration of fluidic transport and positioning with other analysis techniques may lead to highthroughput analysis systems.
Electrical Transport, Sorting, and Characterization. The anatomy of single cells (i.e., phospholipid membrane, ion channels, etc.) allows for interesting interactions between cells and electric fields. Again the ability to fabricate channels with integrated electrodes provides fertile ground for new and improved manipulation and analysis at the cellular level. Several manipulations of single cells in microdevices have been demonstrated, including cell fusion, trapping, transport, and separation.
Dielectrophoresis. The principle of dielectrophoresis is central to many of these devices (see Fig. 5.8). Dielectrophoresis arises from the polarization of a particle in an electric field. If the cell is less polarizable than the surrounding media, the cell will move away from high-field regions (i.e., negative dielectrophoresis). Conversely, if the cell is more polarizable than the surrounding media, the cell will move toward areas of high field (i.e., positive dielectrophoresis, as shown in Fig. 5.8a. In highly conductive solutions (typical of cell culture solutions) the force is always repulsive (negative dielectrophoresis) (Fuhr and Shirley, 1995). Based on this concept, one can design electrode geometries and arrangements capable of trapping single cells (see Fig. 5.8b) or other cell manipulation functions. Many groups have demonstrated sin- gle-cell manipulation using dielectrophoresis. Several functions have been demonstrated, including cell trapping (Fuhr et al., 1992; Schnelle et al., 1993), cell sorting (Washizu, 1990; Kaler et al., 1996), cell transport (Masuda et al., 1987; Hagedorn et
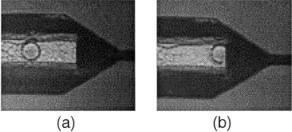
104 |
Microfabricated Fluidic Devices for Single-Cell Handling and Analysis |
al., 1992), and cell characterization (Fuhr and Shirley, 1995; Chan et al., 1998). A good review of cell motion in time-varying fields is given by Fuhr et al. (1996).
Cell Fusion. Another cell-handling operation of importance to modern biology is cell fusion. Masuda et al. (1987) developed a microfabricated electrical cell fusion device over 10 years ago (see Fig. 5.9). The device was fabricated using patterned UV resin as a mold to form silicon rubber microchannels. More recently, Lee et al. (1995) fabricated a similar device using polyimide as the channel material. In both devices, individual cells are brought together from two separate channels and positioned at the electrodes for fusion.
Electrical Impedance. Ayliffe et al. (1998) have used microfabricated devices to directly measure the electrical impedance of single toadfish RBCs and human neutrophils. The devices are similar in geometry to the cell fusion devices described above. Electrical impedance measurements were obtained over a frequency range of 100 Hz to 2 MHz and area-specific membrane capacitances (0.98 F/cm2, neutrophils; 1.59 F/cm2, toadfish) were estimated using an RC circuit.
Magnetic Sorting. The ability to tag cells with magnetic microparticles and subsequently separate them is not new. Recently, however, several groups have demonstrated magnetic-based separations in microfabricated devices. Telleman et al. (1998) describe a device that separates magnetically labeled cells from a continuously flowing stream, as shown in Figure 5.10a. The separation efficiencies are related to field strength and flow rates Liakopoulos et al. (1997) built a device with electromagnets integrated under the flow channel, as shown in Figure 5.10b. When the magnet is turned on, magnetic particles are quickly attracted to the magnet. After rinsing, the magnet can be turned off and the cells collected.
Related Work
Several other areas of microinstrumentation research that may play an important role in future single cell analysis systems are discussed next.
F i g . 5.7. (a) Mouse embryo approaching a constriction. (b) Mouse embryo has reached the constriction and is “parked.”

Single-Cell Manipulation and Analysis |
105 |
F i g . 5.8. (a) Dielectrophoresis can be used to transport cells. The direction of travel depends upon the relative polarizability of the cell and the media. (b) Through proper electrode geometry, dielectrophoretic forces can be used to trap a single cell. (After Fuhr et al., 1996.)
Micro NMR. A group at the University of Illinois at Urbana-Champaign (UIUC) has developed a family of technologies that allow nuclear magnetic resonance (NMR) techniques to be applied to sample sizes approaching the cellular scale. Webb, Sweedler, and co-workers have recently shown that high-resolution NMR spectra and images can be obtained using radiofrequency (RF) detectors with diameters as low as 150 m. These used hand-wound solenoidal coils and were shown to give sensitivities up to two orders of magnitude greater than conventional detectors (Olson et al., 1995; Webb and Grant, 1996).
Work on planar microcoil development proceeded in parallel. The first published application of planar, lithographic microcoils, with feature sizes approaching 1 m, was in 1994 by the UIUC microcoil research group (Peck et al., 1994). This was followed by a parallel study on glass substrates, where spectral linewidths of less than 10 Hz were reported using fused-silica capillaries loaded with 5 mM CuSO4 samples placed directly on top of the coil (Peck et al., 1995). Analogous to results obtained at larger size scales (Wu et al., 1994), the spectral linewidth varied as a function of capillary wall thickness, primarily due to the localized magnetic field distortions that resulted from mismatch in the susceptibility of the coil conductor (gold) relative to the surrounding air. Later studies employing planar, lithographic microcoils provided subhertz spectral resolution using the fused-silica capillary-on-coil configuration and a susceptibility-matching fluid (Stocker et al., 1997). This same group later reported on the first integrated application of microcoils, where a monolithic connection of a GaAs-based planar microcoil, a passive impedance-matching network, and a metal semiconductor field effect transistor (MESFET) preamplifier was employed at 300 MHz for application to neutron NMR microspectroscopy at 7.05 T (Stocker et al., 1995, 1996). A separate, single-turn 1.5-cm RF coil was used for excitation. Early results provided a signal-to-noise (S/N) ratio similar to that of the
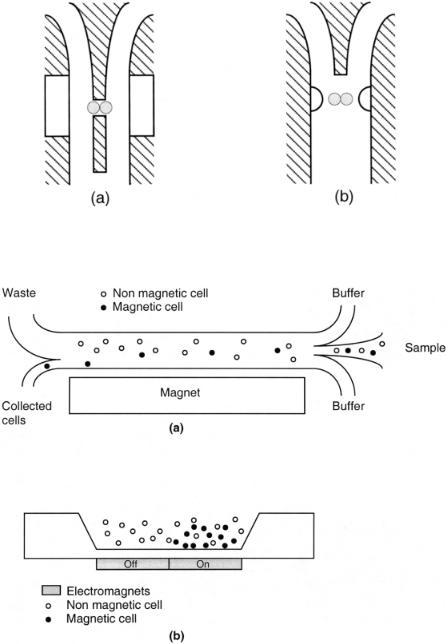
106 |
Microfabricated Fluidic Devices for Single-Cell Handling and Analysis |
F i g . 5.9. (a) Cells are precisely positioned using mechanical and dielectrophoretic effects. (After Masuda et al., 1987.) (b) A similar device relies on dielectrophoretic effects alone to trap and orient cells for fusion. (After Lee et al., 1995.)
F i g . 5.10. Two approaches to magnetic separation devices. (a) Magnetically tagged cells are attracted toward the magnet and collected from a continuously flowing stream. (After Telleman et al., 1998.) (b) Magnetically tagged cells are trapped above the magnet from a flowing stream and later released for collection. (After Liakopoulos et al., 1997.)
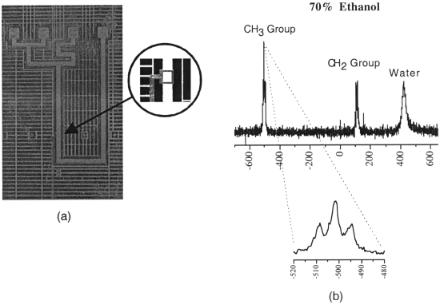
Single-Cell Manipulation and Analysis |
107 |
F i g . 5.11. (a) Microfluidic channel network is integrated with a small NMR receiving coil. The volume under the coil is 30 nl. Channel dimensions are 1 mm 30 µm. (b) High-resolution spectra obtained in the device.
conventional microcoil/preamplifier configuration (in agreement with theory) but poor spectral resolution (presumably due to mismatch in susceptibility of the materials used to construct the coil and amplifier vs. the surrounding air).
The philosophy of miniature total analysis systems ( -TASs) hinges on the integration of multiple chemical processing steps and the means of analyzing their results on the same miniaturized system. Trumbull et al. (1998) have combined microfluidics and micro-NMR in an integrated microfabricated device. Such integration and the ability to precisely control both geometric and material parameters allows for the optimization of multiple operations (e.g., separation and analysis). Figure 5.11 illustrates initial work in this area.
Cell Assays and Electrophoretic Separations. Harrison (1995), Manz (1993), and Ramsey (1994) pioneered capillary electrophoresis in microfabricated devices in the early 1990s. In the following years these groups and others demonstrated a wide range of miniaturized chemical transport, separation, and analysis techniques based on fluorescence and electrokinetic flow and electrophoretic separation principles. The extension of this work toward single-cell analysis is now being explored. Andersson et al. (1997) have demonstrated cell transport and cellular assays in an electrokinetic microchip (see Fig. 5.12). Red blood cells, yeast, and Escherichia coli cells were directed to desired channels within the microchannel